DOI:
10.1039/D4MA00852A
(Review Article)
Mater. Adv., 2024,
5, 9548-9564
Active transfection of genetic materials using cyclodextrin-anchored nanovectors
Received
26th August 2024
, Accepted 18th October 2024
First published on 31st October 2024
Abstract
Gene-based therapy is a sophisticated means for the treatment of various complex diseases like AIDS, cancer, etc., as it resolves the genetic malfunction at the source instead of tackling the superficial symptoms. However, the therapeutic, diagnostic, and theranostic potential of gene-based therapeutic actives such as siRNA, mRNA, pDNA, aptamers, etc. is hindered by physicochemical as well as physiological barriers in the form of insufficient bioavailability, systemic metabolism, rapid renal clearance, inefficient carrier systems, etc. Although advanced carrier systems such as polyplexes, lipoplexes, dendriplexes, hydrogels, polyrotaxanes, etc. are employed to overcome such challenges, their structural configuration results in notable cytotoxicity to induce bio-incompatibility. In this context, strategic integration of cyclodextrins subdues the cytotoxicity by virtue of unique architectural characteristics and allows the fabrication of sophisticated systems for delivery of gene-based therapeutics. Inclusion of cyclodextrins offers benefits like enhanced protection of gene-targeted payloads, compact loading, nanoscale carrier dimensions, biostability, etc. by forming densely packed cargo systems. Cyclodextrins nullify the active cationic moieties to lower in vivo cytotoxicity and improve transfection efficiency across biomembranes. The multi-ligand binding capability of structurally-modulated cyclodextrins avails receptor specificity and gene-targeted therapeutic efficiency. The ability to form reversible covalent linkages allows the fashioning of multi-stimuli responsive supramolecular nanocarriers for a desirable drug release profile. The present review article features cyclodextrins and associated successful applications as the integral components of non-viral nanovectors such as cationic polymers, dendrimers and polyrotaxanes as well as supramolecular assemblies for efficient delivery of RNA-, DNA- and aptamer-based genetic payloads for the achievement of desired treatment outcomes.
1. Introduction
Gene-based therapy encompasses tremendous potential in the permanent treatment of several complex ailments like cancer, neurological problems, autoimmune diseases, etc., as it delivers genetic therapeutics such as siRNA, miRNA, pDNA, aptamers, etc. into the abnormal cells and on-target nuclear components.1–7 Gene-based therapy is also implemented on stem cells to attain genetically modified therapeutic agents as well as gene delivery systems. Such systems employ intracellular signaling networks to modify molecular and cellular activities, enhancing the wound healing mechanisms of the target tissue in cases of skin regeneration, wound therapy and scar formation.8,9 However, the interaction between the genetic payload and systemic biofluids compromises the molecular stability of gene therapeutics. This necessitates the fabrication of sophisticated nanovectors configured from viral sources like lentiviruses, poxviruses, adenoviruses, human foamy viruses, etc., as well as non-viral origins such as cationic polymers, lipids, dendriplexes, stimuli-responsive supramolecular assemblies and polyrotaxanes/polypseudorotaxanes.10,11 As viral vectors elicit significant immunogenic responses and suffer from premature gene expression, an immense focus is placed on the evolution of non-viral vectors for gene delivery.12 Cyclodextrins (CDs) are biocompatible macromolecules of toroidal architecture, with the ability to host various guest molecules via reversible non-covalent interactions for the fabrication of stimuli-responsive delivery systems.13 The inclusion of CDs in non-viral vectors aids to overcome delivery-associated limitations such as cytotoxicity and offer enhancement in gene transfection efficiency. The dual faces of the CD structure along with less hydrophilic cavities provide ample functional zone for further conjugation with peptides, ligands and genetic cargo to impart targeted and controlled gene delivery systems.14 CDs also intensify the proton-sponge effect in non-viral nanovectors to elevate the transfection efficiency across the targeted biomembrane.15 Furthermore, CD-based constructs possess a huge prospect in the development of fluorescent probes, diagnostic assemblies as well as theranostic systems to further augment gene-based therapy.
2. Cyclodextrins
CDs are extensively studied in the pharmaceutical industry due to their potentially superior drug delivery characteristics for the treatment of various cardiological illnesses, neurological maladies, oncological disorders, etc.16–20 CDs are cyclic macromolecular oligosaccharides chiefly constructed of six, seven, or eight α-D-glucopyranose units, named α-CD, β-CD and γ-CD, respectively.21,22 The rings are linked via α-1,4-glycosidic linkages to fashion into a toroidal architecture with a relatively more hydrophobic cavity and hydrophilic exterior, a primary face and a secondary face, respectively, as depicted in Fig. 1.23,24 Such structural features allow the formation of inclusion complexes where CDs encapsulate hydrophobic therapeutics and improve physicochemical properties such as solubility, permeability, stability and bioavailability.25–28 Different CDs possess different dimensions of the hollow space and the conic configuration provides stability to various biomolecules in physiological media by enclosing the molecules and shielding them from non-specific interactions. The carbohydrate composition along with the multiple hydroxyl groups provides the possibility of further chemical modifications like RVD peptide conjugation, linkage to PEI (polyethyleneimine), PLL (poly(L-lysine)), PAMAM (polyamidoamine), PEG (polyethylene glycol), disulfide linkers, etc.29 CDs are versatile carriers, fashioned into several sophisticated formulations like nanoparticles,30 nanosponges,31 stimuli-responsive polymers,32 dendrimers,33 hydrogels,34etc. to achieve an enhancement in therapeutic control. CDs are capable of encapsulating an extensive range of synthetic drugs like doxorubicin (DOX),35 paclitaxel,36 camptothecin,37 levodopa,38 acyclovir,39 as well as large-sized herbal actives like curcumin,40 resveratrol,41 baicalin,42etc. The ability of CDs to conjugate with peptides and proteins,43 nucleic acids,44 carbohydrates,45 and steroids46 to offer flexible therapeutic delivery. The versatile binding potential allows conjugation with receptor ligands for the fabrication of receptor-specific delivery systems. Additionally, the structural incorporation of agents into the CD cavity along with binding to the dual faces of the structure allows multi-drug treatment as well as combinational therapy.47,48 The ability to form reversible covalent bonds with its guest molecules enables site-specific drug release, a desirable duration of action (>48 h) and a sustained release profile. Moreover, appropriate modulation of the chemical structure imparts the desired in vitro and in vivo bio-stability in addition to the longer shelf-life of the formulation.49,50 On the other hand, cyclodextrins classified as generally-recognized-as-safe (GRAS) need to be used carefully as factors such as the number of glucopyranose units, the chemical nature of the substituents, the degree and pattern of chemical substitutions, the HLB value, the applied concentration, the duration of exposure, the presence of serum components, the density of the cells, etc. contribute to the hemolytic attributes of the overall drug delivery complex.51,52 CDs in controlled concentrations and complexed forms such as 2-hydroxypropyl-β-cyclodextrin (HP-β-CD), sulfobutylether-β-cyclodextrin (SBE-β-CD), hydroxypropyl-α-CD (HP-α-CD), etc. are extensively reported as safe for use.53,54 Approved as excellent biomolecules, CDs are globally accepted as pharmaceutical excipients and are extensively explored in modern healthcare as a testament to exceptional biocompatibility, non-immunogenicity, low toxicity and high biological availability.55–57 This encouraged the scientific community to study their potential as non-viral gene carriers and deliver therapeutic genetic materials such as siRNA, miRNA, mRNA, pDNA, aptamers, etc. through different CD-based constructs.
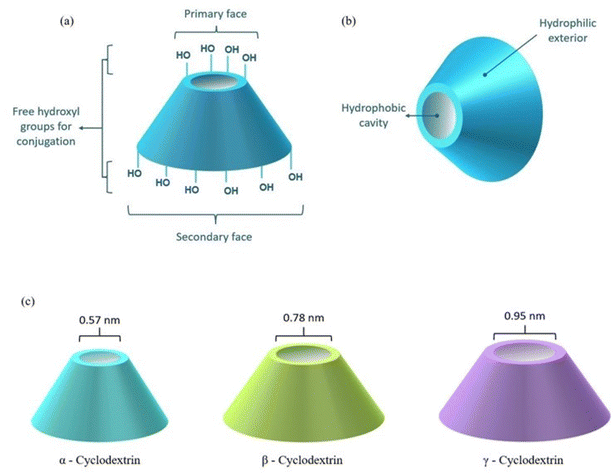 |
| Fig. 1 Toroidal architectures of cyclodextrins: (a) primary and secondary faces of cyclodextrin with free hydroxyl groups for potential structural modification with different groups, (b) the less hydrophilic cavity and hydrophilic exterior of cyclodextrin and (c) the cavity size of different cyclodextrins. | |
3. Challenges in the delivery of genetics
Several extracellular and intracellular barriers hinder the transportation of genetic material like pDNA, siRNA, miRNA, etc. from reaching the site of action and performing gene corrective responsibilities. The epithelial cell membranes, linings along with the extracellular matrix around the cells obstruct the genes from directly accessing target cells. The abundant extracellular nucleases present in the systemic circulation, as well as the extracellular matrix, rapidly deteriorate the free and unprotected genetic material.58 The phagocytic Kupffer cells in the liver and the macrophages of the spleen eliminate the gene-loaded carriers from the bloodstream via renal clearance.59,60 Additionally, due to the hydrophilic nature of naked genetic material and its anionic charge, almost no intracellular uptake occurs due to the poor interaction with the cellular membrane. The passage of genetic material through the cellular membrane is restricted unless their entry is assisted by the generation of transient holes or through various cell uptake processes like endocytosis, pinocytosis, or phagocytosis.61,62 On permeation of the biomembrane, the slow and inefficient movement of the naked genetic material across the protein-filled cytoplasmic matrix prompts insignificant gene expression.63 The genetic therapeutics are further prone to undergo hydrolytic action by hydrolases present in the lumen of intracellular lysosomes. The nuclear envelope represents another hindrance as the double membraned structure embedded with nuclear pore complexes largely regulates the transport across the nuclear membrane and acts as one of the rate-limiting factors in transportation of gene-based therapeutic actives.64 As a consequence, different types of viral and non-viral vectors are required for gene delivery. Viral vectors provide enhanced transfection efficacy but are immunogenic in nature due to viral origins and the immune responses are suppressed to attain transgenic expression.12,65 Systemic administration of viral vectors triggers innate as well as adaptive immune responses against the viral components and gene segments, lowering the efficiency of gene payload. Non-viral vectors display insignificant immunogenicity and cytotoxicity, but exhibit low transfection efficiency.66,67 Such complications in the delivery of gene-based therapeutics necessitate the development of capable carrier systems to achieve high in vivo stability, sufficient transfection efficiency, and low cytotoxicity, and establish effective gene-based therapy.
4. CD-based nanocarrier systems for gene delivery
CDs are frequently employed as one of the core components in gene delivery systems, where the dual faces of the molecule provide the foundation for the addition of various units like glucuronylglucosyl units, RVD peptides, fluorescent moieties, PAMAM, PEI, PLL, etc. CDs enhance permeation of the cargo across biomembranes, potentially preventing the genetic material from non-specific interactions with biological media.68 Incorporation of CDs in polymers,69 dendrimers, supramolecular assemblies, etc. imparts beneficial characteristics to the entire system by acting as a linker or modular component, hosting small molecules, lowering immunogenicity, permeating biomembranes, etc. Although CDs directly interact with the genetic cargo to form ‘pure CD-vectors’, several other studies reported the linkage between the genetic cargo and different complex systems, where CDs played a crucial role, provided carrier stability and ensured endosomal escape of the delivery design.70,71
Cationic polymers are extensively employed in gene delivery as the positively charged amine moieties on the active surface allow electrostatic interactions with negatively charged genetic materials and undergo structural condensation to emerge as gene-loaded polymeric condensates called polyplexes.72,73 Polyplexes provide safety to the genetic material, possess nano-dimensions under 200 nm and offer a desirable storage stability with a zeta potential above 30 mV.74,75 The osmosis-driven proton sponge effect to disrupt the membranes of endosomal vesicles delivers the cargo into the intracellular spaces. However, real-life applications of cationic polymers are limited due to low efficiency of cellular uptake, non-biodegradability, and low biocompatibility.76 As depicted in Fig. 2, the structural fabrication of CDs with cationic and anionic/nonionic groups results in the formation of amphiphilic CD-based constructs. The assemblies resemble cationic polymers as they complex with negatively charged genetic materials to directly self-assemble into polyplexes without any significant in vivo cytotoxic impact.77 Gonzales et al. reported the premier study of β-CD conjugation with cationic polymers for effective transfection of pDNA in the year 1999.78
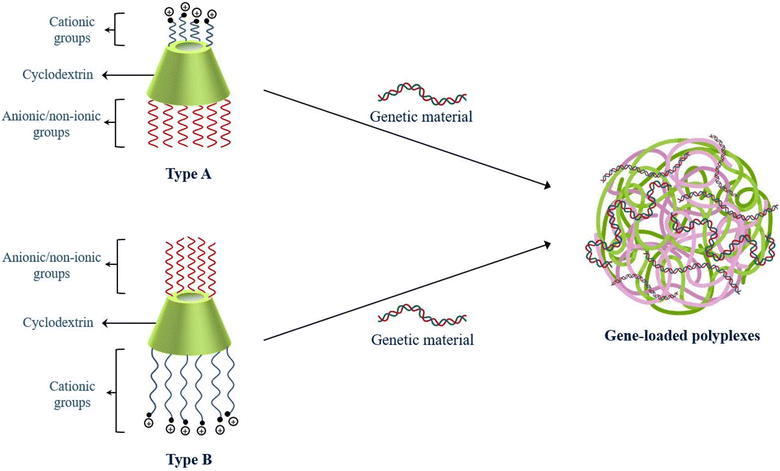 |
| Fig. 2 Amphiphilic cyclodextrin constructs (types A and B) for encapsulation of genetic material and self-assembly into polyplexes. | |
Cationic dendrimers of PAMAM, PEI, PLL, etc. also complex with genetic fragments through terminal amine functional moieties and bind to glycosaminoglycans on the cellular surface for desirable gene transfer. The proton-sponge effect of dendrimers emanating from the hemolytic activity, liposomal membrane-disruptive effect and intracellular distribution, in addition to the superior structural configuration, enhances gene transfer in the target cells.79 However, the use of dendrimers is hindered due to the direct proportionality between dendrimer generation and cytotoxicity.80–82 Significant gene transfer activity is exhibited by high generation dendrimers, but the cytotoxicity intensifies with the increasing number of primary amine terminal zones. In vitro cytotoxicity studies report a tolerable bio-acceptance of lower generation dendrimers, but display a lower transfection efficiency.83 Moreover, the clinical applications of commercially accepted PEI are challenged due to charge-driven binding with intra- and extracellular components.84 As reported in a study, the ED50 value for linear PEI is 4 mg kg−1 in BALB/c mice, severely limiting its usage at higher concentrations.85 The cytotoxicity was known to be directly tackled by altering the linear PEI with CD carbohydrates.86,87 The introduction of cationic-modulated CDs to the free amino terminals of dendrimers (Fig. 3) reduces the cytotoxicity and further enhances the delivery characteristics to enable leak-proof encapsulation of even small genetic molecules like siRNA, ligands, and encapsulation of drugs like DOX, sorafenib, etc.88,89
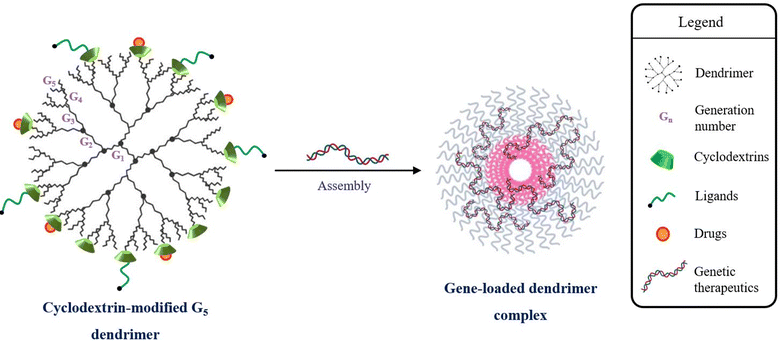 |
| Fig. 3 Cyclodextrin-modified cationic ends of G5 dendrimers for reduction of cytotoxicity and formation of gene-loaded dendrimer complexes. | |
CDs provide the core for accommodation for a variety of molecules and facilitate host–guest interactions to yield supramolecular assemblies. This forte is employed for the fabrication of gene delivery systems as the dual faces of CDs combine with cationic molecules, polymeric strands, proteins as well as peptidyl ligands and further host different guest molecules like adamantane (AD), azobenzene (Az) and ferrocene (Fc).90 AD possesses a suitable size and hydrophobicity to form a desirable host–guest interaction within the CD cavity. Modulated AD groups improve the cationic density of the system and greatly improve the transfection efficiency. PEGylation of AD increases the aqueous solubility of polycations and enhances the bioavailability of the supramolecular gene cargo assemblies.91 Hosting Az and Fc into the CD cavity imparts sensitivity to stimuli such as light, reactive oxygen species (ROS) and intracellular enzymes like glutathione (GSH).92–97 The stimuli-responsiveness to the cargo systems is provided by CD-supramolecular assemblies and presents site-specific therapeutic release as shown in Fig. 4.
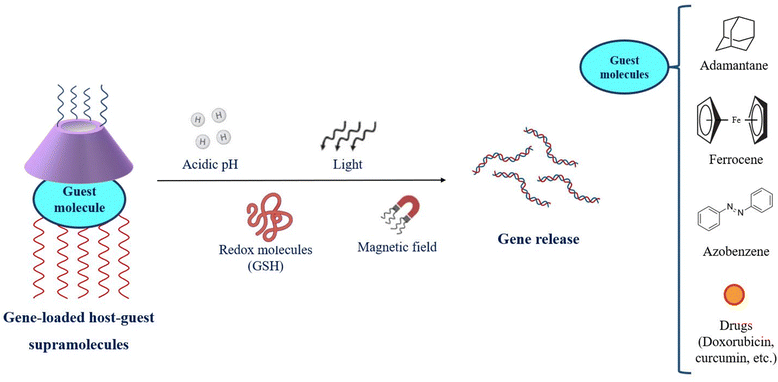 |
| Fig. 4 Stimuli-responsive release of genetic therapeutics from cyclodextrin-based host–guest supramolecular constructs. | |
CD-based polyrotaxanes/polypseudorotaxanes are supramolecular constructs where liner polymeric strands thread through the central cavity of multiple CDs and form mechanically interlocked configurations.98 These configurations are physically flexible in comparison with the traditional polymers and confer additional mechanical stability of the delivery systems. According to the studies, the threading of linear polymers like PEI with CDs reduces their cytotoxic nature and elevates biocompatibility.99 Additionally, the free mobility of CDs provides efficient grafting of gene-binding cationic elements and improves the stability of gene-loaded assemblies. Furthermore, polypseudorotaxanes based on CDs with cationic polymers are used to design controlled release gene-delivering hydrogels as the shedding rate of CDs from the backbone of linear polymers is dictated by their de-threading rate, and the volume of biological fluids stimulates a sustained gene release profile and a lower dosing frequency.100,101 In this manner, the incorporation of CDs potentially enables larger therapeutic frameworks with proficient applications in gene-targeted therapy (Table 1).
Table 1 Advantages of CD-hybrids for genetic delivery
CD-hybrids for the delivery of |
Advantages of CD-hybrids over non-CD based systems |
Ref. |
RNAs |
Effective gene silencing and targeted delivery of siRNA-conjugated CDs |
102,120
|
Enhanced gene knockdown using siRNA-β-CD in comparison with naked siRNA |
103
|
The siRNA–CD complex delivery system exhibited a consistent 85% reduction in CLTC, CAV1 and PAK1 protein expressions and indicated the ability to block key target endocytic pathways. |
104
|
Serum stability of the CD-siRNA was observed |
108
|
Extended protection against enzymatic degradation by nucleases |
109
|
Stimuli-responsiveness, site-specific release and increased therapeutic effectiveness |
111
|
Improved loading of the therapeutic cargo |
117
|
DNA |
Reduction in the cytotoxicity and immunogenic responses of several vector formulations |
123
|
Improved gene stability and transfection ability |
Targeted and safe DNA transportation in vivo |
125–128
|
Stimuli-responsiveness to pH, light, redox and magnetic field is imparted to carriers by CD integration |
Synergistic therapy |
129
|
Development of CD-based theranostic systems for effective gene delivery |
130
|
Aptamer |
Overcomes the instability and conformation flexibility of the aptamer and provides synergistic profiles |
131
|
Stimuli responsive release was achieved |
132
|
Surface engineering with the help of CD molecules with theragnostic applications |
133 and 134
|
Fabrication of high sensitivity and specificity biosensors |
135
|
5. CD-hybrids for RNA delivery
Several studies reported the incorporation of CDs in polyplex structures to obtain sophisticated RNA carriers. Minnaert et al. designed luciferase siRNA-conjugated CDs (CD-ADM70) to develop a nanoparticulate gene-based therapy system against peritoneal carcinomatosis. The siRNAs were conjugated with thiourea segments present in the polycationic amphiphilic CDs to form positively charged systems and effectively interact with negatively charged cell membranes for subsequent cellular uptake. During bioluminescent studies performed on the SKOV-3 human ovarian cancer cell line, administration of 10 pmol CD-ADM70 caused an 85% reduction in luminosity compared to the control group and suggested the insignificant impact of CD-conjugation on the gene-suppressive activity of siRNA. Furthermore, an enhanced gene silencing ability of CD-ADM70 was reported by SKOV-3 cell line studies as 80% downregulation of luciferase gene was observed in the treatment group. Similarly, nebulized CD-ADM70 demonstrated an ∼80% downregulation of luciferase genes, indicating the robust transfection ability and stability of the polyplexes even after exposure to a high-pressure dosing system.102 Malhotra et al. directly conjugated β-CD with the sense strand of siRNAs by a bioreducible disulfide linkage for targeted silencing of overexpressed luciferase and PLK1 genes in cancer cells. Furthermore, ligand systems of adamantyl-conjugated RVD peptides were incorporated into the cavity of siRNA-conjugated CDs to generate polyanionic constructs. The constructs were charge-neutralized using polycationic chitosan to develop ligand-directed CD-siRNA nanoparticles with dimensions of ∼100 nm. Cell culture studies performed in the U87 human brain cancer cell line reported a 70% reduction of PLK1 gene expression compared to the untreated group, whereas similar studies performed in the DU145 prostate cancer cell line reported a 75% reduction. A luminescent intensity study performed on luciferase-overexpressing PC3-Luc cells reported a luminescence of 1500 units by CD-siRNA-treated cells compared to the 7500 units of scrambled siRNA cells and reflected a gene knockdown effect of 80%.103 In a related study performed by Manzanares et al., a β-CD-based multivalent amphiphile, AMC6, was conjugated with different siRNAs as transfection vectors to knock down SCR, CLTC, CAV1 and PAK1 protein expressions. The AMC6 structure possesses 28 cationizable centers as the primary ring surface of β-CD was linked to seven tetraethyleneimine linkers, each containing four protonatable nitrogen atoms. This allowed stable interactions with negatively charged siRNAs and rapid self-assembly into nanoparticles with an average size of 110 nm. Fluorescent studies performed on the C6 rat glioma cell line, GL261 rat glioma cell line, U87 human glioblastoma cell line and T98G human glioblastoma cell line revealed a maximum fluorescence of nearly 4000 RFU, 4500 RFU, 5100 RFU and 5000 RFU, respectively, suggesting the effective siRNA transfection ability of the nanoparticles after an incubation time of 8 h. At a strength of 100 nm, the siRNA delivery systems exhibited a consistent 85% reduction in CLTC, CAV1 and PAK1 protein expressions during 72 h cell line studies and indicated the ability to block key target endocytic pathways.104
Zhang et al. co-delivered siRNA and DOX by the fabrication of nanoparticles from carboxymethyl-β-CD (CM-β-CD) grafted trimethyl chitosan (TMC). Here, carboxymethylation of β-CD improved the solubility as well as hemocompatibility of the siRNA carrier. In vitro studies revealed that a 10 mg mL−1 formulation induced an insignificant hemolysis of 0.76%. The moiety also underwent dehydration with the amino groups of the TMC chain to form a stable and safe graft polymer. At a strength of 1 mg mL−1 siRNA and 10 mg mL−1 DOX, the A549 cells exhibited an effective 10% cell viability, indicating the in vitro effectiveness of the formulation.105 Evans et al. employed an amphiphilic CD-based vector for the delivery of siRNAs to prostate cancer cells and incorporated a folate-targeted fusogenic peptide, GALA, for ligand-specific uptake of the therapeutic siRNA. The primary face of CD provided the base for lipophilic C8 chains, whereas the secondary face was linked to cationic groups for conjugation with different siRNA molecules. Insignificant cytotoxicity levels were observed in healthy cells as a 95% survival rate was reported during cell viability studies. Cell culture studies performed on PC3 cancer cells revealed an effectively reduced relative luciferase expression by 75% and suggested a significant uptake of carrier vectors as well as effective gene silencing activity. Similarly, selective mRNA-silencing was also noted as 50% NRP1 and 45% ZEB1 mRNA suppression were reported compared to the 100% expression observed in control groups. Significantly, the treatment of 3D spheroidal tumors with the nanoparticles resulted in lower metastasis, reduced infiltration in the Matrigel layer and formation of compact non-invasive colonies.106
Kathleen et al. modified CDs with dilysine on their primary face for interaction with siRNA moieties. On self-assembly into nanoparticles, the anionic siRNA was encapsulated within the cationic dilysine–CD assembly for safe and stable gene delivery. CDs also availed host–guest interactions with AD-linked PEG strands modified with anisamide as a tumor-targeting ligand. MTT assays in cancer cell lines reported a cell viability of >80% 24 h post-transfection, indicating the safety of the CDs. After incubation for 48 h, a relative gene expression of ∼75% in the test group compared to 100% in the control group revealed the effectiveness of the system to safely and effectively deliver siRNA to the target cells.107 Evans et al. prepared amphiphilic CDs and further modified them with folate-targeting ligands for assembly into siRNA encapsulated nanoparticles.108 The charge neutralizing ability of the CD moiety to result in an optimized siRNA carrier was reflected by an 8 h serum stability test as well as cell viability tests in healthy cells. In vivo studies performed on male BALB/c mice reported a high t1/2 of 14.61 h, a high AUC of 12.24 ng h mL−1 and a low clearance of 2.01 L h−1, further proving the in vivo stability as well as activity of the design. Gooding et al. modified amphiphilic β-CD derivatives with rabies virus glycoprotein (RVG) for glioblastoma-specific delivery of siRNA. In the presence of siRNA, the modified β-CD molecules self-assembled into nanomicelles with siRNA strands encapsulated between the β-CD layers. In vitro analysis revealed extended protection of siRNA for 24 h against the enzymatic degradation by nucleases. Additionally, incubation of the nanomicelles with U87 human glioblastoma cells caused a 27% reduction in endogenous glyceraldehyde 3-phosphate dehydrogenase (GAPDH) mRNA expression, demonstrating a potential improvement in glioblastoma therapy.109 Chen et al. constructed a novel carrier system using CDs for an innovative approach for co-delivery of siRNA, aptamer and the antitumoral drug sorafenib for combined anticancer activity. Patterning RCT-synthesized gene products on amino-CD-modified (amCD) DNA probes promoted the formation of nanoparticles as the positively charged amCDs reshaped the RCT structures via electrostatic interactions with RNA strands to form porous nanospheres. The less hydrophilic cavity of CDs further allowed host–guest encapsulation of insoluble sorafenib to treat the tumor tissue and suppress further growth of the tumor. 24-Day in vivo studies performed on tumor bearing nude mice reported a tumor volume of 300 mm3 compared to the volume of 1200 mm3 in control groups. The tumor xenografts collected from the treatment group showed a depletion in epithelial cell adhesion molecule (EpCAM) expression levels, whereas the phosphorylation of extracellular signal-regulated kinase (ERK) and cRAF was consistently restrained to highlight the effective siRNA transportation and gene silencing activity of the therapeutic system.110
A distinctive ability imparted by the application of CDs is the establishment of stimuli-responsive supramolecular delivery of genes due to the ability to form reversible host–guest systems. This property demonstrates great potential in site-specific release and increases the therapeutic effectiveness. Li et al. fabricated strands of hyaluronic acid (HA) with α-CDs for conjugation with siRNA, where Az-modified diphenylalanine derivatives (trans-G) acted as linkers between CDs and siRNA to achieve trans-G/HA-α-CD/siRNA ternary supramolecular nanoassemblies. Photosensitivity was imparted to the system as 365 nm UV irradiation triggered conformational changes in the trans-G structure to weaken its α-CD association stability and lead to carrier disassembly for the release of siRNA. As confocal laser fluorescence microscopy confirmed the photo-responsive cellular delivery of siRNA in the A549 human lung adenocarcinoma cell line, GAPDH expression levels lowered by 55%, suggesting effective siRNA delivery and gene-silencing efficiency of the formulation. Further evidence of siRNA activity was provided as abnormal gene expression resulted in a 50% growth inhibition of the tumor cells at a concentration of 80 mM after UV irradiation.111 Similarly, Zhang et al. designed novel photo-responsive supramolecular constructs of CD–AD to suppress firefly luciferase siRNA expression. β-CD hosted amantadine groups conjugated with the photo-sensitive linker phosphoramidite, which was terminally linked to the 5′ end of siRNA. Studies performed on HEK293T cells reported a 6-fold reduction in luciferase expression after a 3 min exposure to UV light. In the absence of β-CD, the gene expression was noted as nearly 96% in the absence of UV light, indicating the introduction of photo-modulation by β-CD incorporation. On employing HA-conjugated CD, the pre-UV exposure leakage was notably reduced with improved cellular delivery and photo-modulated gene-suppression of the endogenous Eg5 gene was also achieved.112
Wang et al. coated gold nanorods (GNR) with CD-grafted PEI for dual loading of docetaxel (DTX) and siRNA-p65 and achieve a multi-active host–guest-based chemotherapy. Infrared-triggered hyperthermia effectively drove the cytosolic release of siRNA and DTX as displayed by confocal laser scanning microscopy (CLSM) images. Without laser treatment, the internalized siRNA was entrapped within the lysosomes for 12 h, whereas exposure to an IR laser for 3 min promoted siRNA escape to enhance the therapeutic efficiency of the active carrier. In vivo studies performed on Wistar rats reported a tumor volume of ∼400 mm3 in the siRNA-loaded formulation compared to the ∼1350 mm3 and 800 mm3 of PBS- and GNR-treated groups, respectively, indicating the in vivo efficiency of siRNA-p65 delivery along with potential therapeutic effectiveness.113 Xiong et al. developed a supramolecular nanoparticulate system for hepatoma-targeted co-delivery of oligoRNA and DOX. Polylysine (PLL) polymer was conjugated to the smaller face of β-CD and DOX was encapsulated within the CD cavity via host–guest interaction.114 The resultant polycationic framework was condensed with oligoRNA to form supramolecular nanoparticles and further coated with HA for CD44-mediated endocytic targeting. pH-responsiveness behaviour was observed during in vitro studies as to the formulation as 47.3%, 37.4%, and 31.8% of the drug was released at pH 5.0, 6.5, and 7.4, respectively. CLSM images revealed high fluorescence of free oligoRNA in the cytosol of MHCC-97H cells and HepG2 cells, suggesting successful transportation and pH-responsive release of oligoRNA along with DOX. Similarly, Xiong et al. fabricated a pH-responsive supramolecular carrier for synergistic release of miR-122 as well as DOX for targeted hepatic activity.115 The β-CD-based star copolymer nanoparticles contained a core of polyCD-conjugated PDMAEMA for host–guest interaction with DOX as well as attachment to miRNA. pH-responsiveness was noted as 94% miRNA was released at pH 5.0 compared to 60% at pH 7.2, whereas CD incorporation further delayed DOX release by 6 h due to the decreased drug diffusion from the CD core.
The application of dendrimers to impart benefits is hindered due to challenges like cellular toxicity and low transfection rates into target cells. Arima et al. reported a study conjugating β-CD with polyamidoamine dendrimer for an enhanced transfection ability of pDNA in the year 2011.116 The incorporation of CDs into the dendrimer compensated free amine groups to reduce the cationic charge on the carrier and lower the toxic impact on cells. CDs also facilitate the release of genetic material after endosomes after endocytic uptake, thus overcoming the low transfection efficacy problem. This strategy was employed by Qiu et al. as they conjugated PAMAM dendrimers with β-CD and entrapped gold (Au) nanoparticles in the assembly for enhanced delivery of siRNA. The amine groups of G2 dendrimers interacted with β-CD fractions, which further bonded with two cationic siRNAs, namely B-cell lymphoma 2 (Bcl-2) and vascular endothelial growth factor (VEGF), for silencing the genes in cancer cells. β-CD also improved the therapeutic loading due to tight siRNA compression into the resultant polyplexes. The safety of the CD-dendrimers was confirmed as the MTT cytotoxicity assay on U87MG cell lines reported >80% cellular viability even at a higher drug concentration of 2000 nM. Bcl-2 and VEGF expression studies reported a 25% expression in vector exposed U87MG cells compared to the 100% expression in naked-siRNA exposed cells, indicating the heightened gene transfection of siRNA by the CD-vector system.117 Similar observations were reported by Hayashi et al. as they developed siRNA polyplex delivery with hepatocyte-specific action for anti-amyloidosis therapy.118 The lactose appended G3 dendrimer was conjugated with α-CD for loading siTTR siRNA. The role of α-CD was established as the carriers without CD displayed low gene silencing activity against the CD-containing polyplexes. In the absence of α-CD, the polyplexes circumvented the high endosomal escape activity to elicit insufficient interactions with phospholipids, resulting in transfection driven solely by the proton sponge effect of the dendrimer. The CD-conjugated dendrimers displayed more safety in the HepG2 cell line as compared to lipofectamine 2000 carriers with a 3-fold increased cell survival rate even at a 100 nM concentration. Furthermore, 50% gene silencing activity reported during in vivo studies using BALB/c mice indicated the vital impact of α-CD on the carrier. Modified β-CD groups such as glucuronylglucosyl-β-CD (GUG-β-CD) interact with endosomal membranes to further improve the endosomal escape of the siRNA complex post membrane disruption.119
Mohammed et al. reported the utility of GUG-β-CD conjugation with G3 dendrimers as siRNA carrier systems. α- and β-CDs were linked to the dendrimers via a GUG linker fraction and interacted with siGL3 siRNA to evaluate the gene silencing efficiency in vitro. A ∼80% suppression of luciferase activity noted after incubation for 23 h indicated the effective delivery of a gene-based therapeutic active, i.e., siRNA, by the CD-modulated system. Fluorescence tests to detect intracellular distribution revealed that a considerable number of assemblies escaped endosomes after uptake, whereas the assemblies released the gene-based therapeutic active in the cytoplasm. A compilation of results suggested enhanced endosomal escape ability and siRNA releasing properties of GUG-β-CD systems.120 Further, Mohammed et al. conjugated a DOX-loaded G3 PAMAM dendrimer with GUG-β-CD for complex formation with siGL2 and siPLK1 siRNAs for effective antitumor therapy. In vivo studies performed on BALB/c mice reported a tumor volume of ∼375 mm3 and ∼600 mm3 in the siPLK1- and siGL2-vector groups, respectively, compared to ∼650 mm3 in the control group after 15 days of injection.121
Polyrotaxane structures are potential RNA carriers due to their targeted delivery characteristics and gene protective abilities. Gocke et al. employed α-CD-threaded polyester polyrotaxanes to deliver GFP siRNA and studied their in vitro gene transfection ability. The rotaxane α-CD further provided the base for modification with DMEDA to achieve a stronger linkage with siRNA due to free rotatability and form dense nanoplexes with significant gene loading. The assembly displayed significant biosafety with an 80% cell viability in normal HeLa cells, notably greater than that of the standard transfection agent branched PEI. Gene silencing assays on HeLa cells reported a 50% gene silencing efficiency of the optimized formulation compared to the 100% of the control group, and CLSM images showed macrophage-driven transfection complex internalization within 4 h of the treatment.122
6. CD-hybrids for DNA delivery
CDs reduce the cytotoxicity and immunogenic responses of several vector formulations and hence hold immense potential to deliver DNA, just like potential RNA delivery. Elsana et al. improved the gene stability and transfection ability of polyplexes using CM-β-CD as the non-viral vector. CM-β-CD enhanced the pDNA condensation as reflected by a 45.20% inclusion rate and provided protection to the pDNA in the DNase I environment even at low concentrations as revealed by gel electrophoresis. The polyplexes significantly transfected the COS 7 and SH-SY5Y cell lines as well as increased the transfection against the commercial transfection reagent TransIT-LT1. In addition to polyplexes, liposomal systems incorporated with CDs also show promise in targeted and safe DNA transportation. Structural integration of less polar CDs results in non-specific interactions with the cell membrane during ingestion by endocytosis rather than sole electrostatic interactions to display an enhancement of gene transfection in liposomal vectors.123 Elsana et al. formulated cationic lipoplexes with the aid of CM-β-CD for efficient condensation of pDNA into stable nanomicelles of dimensions under 160 nm. Gel electrophoretic studies revealed sufficient protection for DNase I enzyme, indicating potential in vivo stability with adequate condensation of pDNA into the nanoparticles. A transfection efficiency of above 40% was consistently observed in CD-based groups compared to <30% for the control formulation during in vitro studies on Sh-SY-5Y cells. The safety of the formulation reflected by >80% during COS7 cell line studies ensured the non-toxicity of the CD-based formulation.68 Similarly, Štimac et al. merged β-CD into the liposomal design by conjugating the primary rim of CDs with hydrophobic n-dodecyl chains, whereas the secondary rim was linked to hydrophilic oligo(ethylene glycol) substituents. The resultant complex was fused within the phosphatidylcholine moieties of the liposomal vesicles to bind pDNA via suitable linkers.124 Stimuli-responsiveness to pH, light, redox and magnetic field is assigned to carriers by CD integration.
Zhang et al. designed a pH-responsive supramolecular polymer by conjugation of the poly(β-CD) (PCD) backbone with poly(glycidyl methacrylate) (PGEA) via acetal bonds to generate a cationic PCD-acetal-PGEA carrier assembly. Additionally, β-CD also formed complexes with AD-modified ligands for targeting folate receptors. The resultant supramolecular polymer showed the ability to form polyplexes with both anti-EGFP siRNA and pcDNA3-Luc plasmid DNA. The gene transfection assay performed on 293T, HeLa and MCF-7 cell lines using pDNA as a reporter gene revealed a noteworthy 50% reduction in luciferase expression.125 Similar observations were noted on using pEGFP as a reporter gene during cell culture studies and the siRNA knockdown results demonstrated the effectiveness of multifunctional supramolecular gene delivery assembly to deliver siRNA to cancer cells. Photodynamic nanoplatforms of CD-based supramolecules were developed by Wang et al. for better control over DNA delivery. By modification of cationic PLL with β-CD, photo-responsiveness was incorporated into the systems as CDs hosted TPP-PEG as a water-soluble photosensitizer for synergistic anticancer activity. The CD-constructs are self-assembled into polyplexes, with β-CD-PLL encapsulating pDNA within its cavity via stable electrostatic interactions. CLSM studies performed on HeLa cells reported an intense red fluorescence intensity after 15 min LED exposure compared to the low fluorescence observed before LED exposure, indicating the photo-responsive cleavage of the host–guest molecule to provide a controlled gene delivery.126
The redox-responsive trait was incorporated into the DNA delivery system by Sahoo et al. by bridging β-CD-scaffolded polycationic clusters with redox-sensitive AD-S-S-AD groups. The resultant construct showed great DNA condensing properties by virtue of its four cationic arms and form polyplexes with a significant DNA payload. The polyplexes also exhibited dense structures with particle sizes below 300 nm due to compact intra-scaffold binding. In the presence of 10 mM GSH mimicking the intracellular concentration scenario, a significant fluorescence intensity of 85 RFI was observed for 4 h, whereas the fluorescence was absent in GSH-deprived concentrations. This confirmed the potential applications of CD for redox-triggered gene release.127 Magnetic stimuli-responsive CD-based assemblies for gene delivery were developed by Li et al. as the superparamagnetic iron oxide nanoparticles (SPIONs) were modified with hydrophobic oleyl chains to specifically stabilize within the less hydrophilic α-CD cavity. The CDs were externally conjugated with cationic OEI to form star cationic polymers for stronger binding with pDNA and form α-CD-OEI-SPIONs/pDNA complexes. Transfection efficiency, reflected by luciferase expression, was greatly enhanced on the application of an external magnetic field as the carriers were rapidly attracted towards the cell membrane and sped up sedimentation to aid in membrane permeation. The in vitro studies with exposure to magnetic fields reported a 25% increase in luciferase expression in the MCF-7 cell line. Additionally, the CD-cavities hosted the disulfide-containing Az-terminated branched polymer attached to fluorescent rhodamine groups for a site-triggered fluorescent activity. In vivo studies in zebrafish embryos reported insignificant cytotoxicity along with significant gene transfection efficiency.128 CDs also demonstrate potential for the development of multi-staged inclusion complexes as reported by Ke et al. By conjugating Nur77 plasmid-complexed β-CD-PCL-PDMAEMA with DOX-enclosed β-CD-AD strands, synergistic gene therapy was established based on the host–guest complexation of CDs. The dense packing of the construct imparted a smaller and more stable particle size in comparison with PEI-25k. Moreover, due to the higher cationic charge, the complex exhibited a better pDNA binding ability against the standard PEI-25k. With an improved cellular uptake, it proved as a better gene transfection vector in HepG2/MDR1-Bcl2 cells as a consistent luciferase activity of >107 RLU per mg was reported compared to the variable PEI-25k results. By encapsulating DNA in a self-assembled inclusion complex synthesized by linkage of 2 CD-based polymers and hosting fluorescent probes in the CD cavity, CD-based theranostic systems are developed for gene delivery as well as real-time therapeutic progress.129 Jiang et al. designed targeted and fluorescent traceable supramolecular polyplexes by employing PEGylated CD chains, where the strands interacted with DNA and formed stable genetic complexes for gene therapy, demonstrating the immense scope of CD-based DNA theranostic systems.130
7. CD-hybrids for aptamer delivery
Application of CDs as critical components in various formulations also resolves the delivery challenges of instability and conformational flexibility faced during aptamer transportation. Moreover, CDs encapsulate synthetic actives to provide a synergistic treatment profile. Jiang et al. conjugated β-CD with circular, bivalent aptamers for stable delivery of saporin protein for its antitumor activity. By linking β-CD to the aptamers via linkers, the bivalent genetic material retained high serum stability. β-CD also hosted AD-modulated peptides to introduce antitumor activity as well as enhanced cellular internalization of the system. The resultant suprapolymeric system further encapsulated Au nanoparticles for enhanced cytotoxicity. HeLa cell line studies reported a 3-fold increased fluorescence intensity and a cytotoxicity of ∼40% at a concentration of 75 nM, suggesting safe delivery of aptamers and formulation effectiveness, respectively. In addition to stability, CDs are also utilized to strategize stimuli-responsive release of aptamers via stimuli-sensitive disulfide linkages.131 Shen et al. fabricated β-CD-dependent pH-activated mesoporous silica nanocarriers (MSNs) for the treatment of breast cancer cells using human epidermal growth factor receptor-2 (HApt) aptamers. After capping the MSN pores with β-CD-SH, the opposite face of CDs was functionalized with the aptamer HApt via disulfide bonding. In vitro tests confirmed the pH-stimulated aptamer release as the discharge of equivalent DOX revealed an 80% release within 10 h at pH 4.5 compared to 30% at pH 6.4. At a strength of 500 μg mL−1, the nanoassemblies displayed the potent cytotoxicity of HApt aptamers with a cell viability of 45% in HER2-overexpressing SKBR3 breast cancer cell lines to demonstrate the effective separation of aptamers from the β-CD-SH moiety. Surface modification of nanomicelles with aptamers is also made possible by employing conjugated CDs as linker molecules.132 Li et al. conjugated the aptamer AS1411 to the surface of Pluronic F127 nanomicelles using β-CD-linked PELA block copolymers for enhanced tumor-targeting and nucleolin-mediated cellular internalization of the therapeutic system. During the in vivo studies performed on MCF-7 tumor-bearing mice, an average signal of 550 units in tumor tissue was reported compared to the <200 units reported in other vital organs after a dose of 5 mg kg−1. Average fluorescence signals observed during real-time near-IR fluorescence imaging suggested a better tumor targeting potency of CD-associated aptamer-micelles compared to the non-conjugated ones.133
8. CD-hybrids for aptamer-based diagnostics
Ultrasensitive sensors and diagnostic tools are driven by the concept of conjugation between CDs and aptamers. Hasanzadeh et al. fabricated Au nanoparticles and immobilized DNA-aptamers on the surface using α-CD for the detection of the platelet-derived growth factor-B (PDGF-B) tumor biomarker. The biosensor exhibited a superior sensitivity, a reduced detection limit and high stability for PDGF detection with a linear range of 50 pM–10 nM and an LOD of 50 pM.134 Wu et al. developed a sensitive electrochemical aptamer biosensor for tetracycline determination. The TET aptamer was bound to ferrocene as a signaling section, which was further hosted by the main body of β-CD associated with the Au electrode. In the presence of TET, the aptamer undergoes a configurational change to result in separation from the electrode surface. The accurate detection of tetracyckine in tap water, milk, substrate, etc., enhanced the potential applicability of the HS-βCD-based subject–object recognition technique to develop highly specific and sensitive biosensors was established.135 On similar lines, He et al. also developed a fluorescent assay for the detection of tetracycline using γ-CD as a stabilizer. The triple helix aptamer probe (TAP) formed an inclusion complex with γ-CD via a pyrene dimer to form a probe with a low detection limit of 1.6 nM. Target exposure results in structural changes, leading to separation of the signaling probe from the aptamer and generation of pyrene excimer. γ-CD stabilizes the excimer using host–guest inclusion and stabilizes the signaling performance, to remarkably increase the fluorescence intensity. Owing to the stability imparted by γ-CD, the fluorescence is sustained for an extended duration to give an ultrasensitive, stable, specific and efficient biosensor.136 With the development of α-CD-based polyrotaxanes, Zu et al. utilized aptamers for enhanced magnetic resonance imaging (MRI) contrast agents for tumour-targeted imaging. The α-CD axle around the disulfide-modulated PEG thread bestowed the site for modification with lysine dendrons as the connector for gadolinium chelation as well as AS1411 aptamers. The α-CD axle provided free mobility and enabled the modified AS1411 aptamer with suitable rotation as well as slidability. This facilitated the opportunity for greater interaction with surface nucleolins and the achievement of multivalent targeting for faster accumulation and intratumoral retention of contrast for an extended duration at the tumor site. In the tumor microenvironment of high GSH concentration, the biodegradable disulfide linkage undergoes de-threading and results in non-toxic Gd moieties with easier elimination processes. Cellular images of the in vitro MCF-7 cancer cell line showed a 5-fold increase in signal intensity compared to the control, whereas in vivo MRI studies in tumor bearing mice displayed an evident contrast of 1.5 relative enhancement signal intensity (RESI) after 0.5 h and intensified till 3 RESI till 4 h compared to the consistent 1 RESI of control, indicating the faster accumulation and longer residence time attributed to the α-CD-linked AS1411 aptamer.137
9. Future directions for research
CDs, a class of glucose-linked cyclic molecules, are being widely studied for their role in polyplexes and supramolecular polymers for delivering siRNA, pDNA, and gene therapeutics. Ongoing research aims to enhance the efficiency and specificity of gene delivery, with new CD-based polyplexes showing promising results in pre-clinical models. CDs improve the bio-stability, non-toxicity, biodegradability, site-specificity, and systemic solubility of gene vectors. Future research will focus on incorporating CDs for targeted delivery in cancer therapy and regenerative medicine, utilizing surface modifications to improve therapeutic efficacy. Another area of research is the enhanced delivery of therapeutic genes to the brain, as CDs have shown the ability to cross the blood–brain barrier in animal models, suggesting potential for gene-based therapies in neurological disorder.138–140 CDs are further employed for non-viral neuronal targeting for siRNA delivery, as reported in various experiments.141–143 The ability of CDs to enhance site-specificity and gene delivery efficiency makes them promising candidates for gene theranostics, combining disease diagnosis and treatment through gene therapy. CDs are complexed with receptor-specific moieties to introduce molecular recognition, further improving the precision of gene-based delivery systems.109,144–146 CDs are also being explored to enhance the delivery of CRISPR-based therapies, which use guide RNA and Cas enzymes to target and edit specific genes.147 However, cyclodextrin-based systems are still in early development, and further studies are needed to confirm their safety and efficacy in humans. Research has shown CDs can deliver diagnostic genes, such as imaging reporter genes, to visualize diseases in vivo and to enable personalized therapies in the future.148 CDs are highly sensitive to various molecules, including gases, ions, and biomolecules like proteins, enzymes, lipids, and genes, making them ideal candidates for sensing materials in Internet of Things (IoT) devices. Additionally, they hold potential for low-cost, sensitive diagnostic technology, targeted drug release systems, and future research may focus on IoT-based delivery systems like drug injectors and biomonitoring systems.149–153 Moreover, CDs are used to develop sensors for specific genetic biomarkers, such as mutations and gene expression changes.154 While CDs show promise in IoT applications, more research is required to confirm their full potential and safety. In summary, their unique properties make CDs valuable for future gene delivery, personalized medicine, and IoT uses such as sensing, drug delivery, and biomedical monitoring.
10. Conclusion
Advances in gene-based therapy are constrained by delivery challenges and carrier systems. Cyclodextrins (CDs) are versatile macromolecules with multiple conjugation sites for linkers and genetic payloads. Numerous in vitro and in vivo studies of CD-integrated nanoparticles show enhanced biomembrane permeation and improved transfection efficiency to enable precise gene delivery. Modified CD systems allow for higher surface conjugation with receptor ligands to enable targeted delivery and reduce adverse effects. Combining CDs with cationic moieties in polyplexes, lipoplexes, dendrimers, and supramolecular constructs lowers biotoxicity and enhances in vivo stability. Host–guest interactions, facilitated by covalent linkages with linkers like azobenzene and ferrocene, enable pH-, redox-, light-, and magnetic field-sensitive stimuli-responsive gene release. Additionally, modification of CDs with fluorescent elements enhances probe generation and theranostic assemblies. Given these advantages, CDs are promising for future gene delivery systems and personalized therapies, contributing to real-time monitoring and improved treatment efficacy.
Author contributions
Amey Revdekar: visualization, writing – original draft. Bhagyashree V. Salvi: visualization, writing – original draft. Dr. Pravin Shende: conceptualization, writing – review and editing, supervision.
Data availability
No primary research results, software or code have been included and no new data were generated or analysed as part of this review.
Conflicts of interest
The authors declare that there are no conflicts of interest.
References
- A. Akbari, F. Rahimi, Z. Abbassi Radmoghaddam, S. Honarmand, T. Godary, M. Ghasemi Toudeshkchouei and S. Akbari, β-Cyclodextrins-based nano carriers for cancer therapy, NanoSci. Technol., 2021, 1, 1–11 Search PubMed.
- H. A. Seo, S. Moeng, S. Sim, H. J. Kuh, S. Y. Choi and J. K. Park, MicroRNA-Based Combinatorial Cancer Therapy: Effects of MicroRNAs on the Efficacy of Anti-Cancer Therapies, Cells, 2019, 9, 29, DOI:10.3390/cells9010029.
- G. Mahmoodi Chalbatani, H. Dana, E. Gharagouzloo, S. Grijalvo, R. Eritja, C. D. Logsdon, F. Memari, S. R. Miri, M. Rezvani Rad and V. Marmari, Small interfering RNAs (siRNAs) in cancer therapy: a nano-based approach, Int. J. Nanomed., 2019, 14, 3111–3128, DOI:10.2147/IJN.S200253.
- J. Li, H. Liang, J. Liu and Z. Wang, Poly (amidoamine) (PAMAM) dendrimer mediated delivery of drug and pDNA/siRNA for cancer therapy, Int. J. Pharm., 2018, 546, 215–225, DOI:10.1016/j.ijpharm.2018.05.045.
- T. Li, G. Kang, T. Wang and H. Huang, Tumor angiogenesis and anti angiogenic gene therapy for cancer (Review), Oncol. Lett., 2018, 16, 687–702, DOI:10.3892/ol.2018.8733.
- S.-A. Shu, J. Wang, M.-H. Tao and P. S. C. Leung, Gene Therapy for Autoimmune Disease, Clin. Rev. Allergy Immunol., 2015, 49, 163–176, DOI:10.1007/s12016-014-8451-x.
- B. E. Deverman, B. M. Ravina, K. S. Bankiewicz, S. M. Paul and D. W. Y. Sah, Gene therapy for neurological disorders: progress and prospects, Nat. Rev. Drug Discovery, 2018, 17, 641–659, DOI:10.1038/nrd.2018.110.
- L.-H. Peng, S.-Y. Tsang, Y. Tabata and J.-Q. Gao, Genetically-manipulated adult stem cells as therapeutic agents and gene delivery vehicle for wound repair and regeneration, J. Controlled Release, 2012, 157, 321–330, DOI:10.1016/j.jconrel.2011.08.027.
- P. Shende, H. Gupta and R. S. Gaud, Cytotherapy using stromal cells: Current and advance multi-treatment approaches, Biomed. Pharmacother., 2018, 97, 38–44, DOI:10.1016/j.biopha.2017.10.127.
- G. A. R. Gonçalves and R. de M.A. Paiva, Gene therapy: advances, challenges and perspectives, Einstein, 2017, 15, 369–375, DOI:10.1590/s1679-45082017rb4024.
- Y. Sung and S. Kim, Recent advances in the development of gene delivery systems, Biomater. Res., 2019, 23, 8, DOI:10.1186/s40824-019-0156-z.
- S. Akira, S. Uematsu and O. Takeuchi, Pathogen Recognition and Innate Immunity, Cell, 2006, 124, 783–801, DOI:10.1016/j.cell.2006.02.015.
- S. A. Fahmy, J. Brüßler, M. Alawak, M. M. El-Sayed, U. Bakowsky and T. Shoeib, Chemotherapy Based on Supramolecular Chemistry: A Promising Strategy in Cancer Therapy, Pharmaceutics, 2019, 11, 292, DOI:10.3390/pharmaceutics11060292.
- H. M. Chu, R. X. Zhang, Q. Huang, C. C. Bai and Z. Z. Wang, Chemical conjugation with cyclodextrins as a versatile tool for drug delivery, J. Inclusion Phenom. Macrocyclic Chem., 2017, 89, 29–38, DOI:10.1007/s10847-017-0743-3.
- C. Bienvenu, Á. Martínez, J. L. Jiménez Blanco, C. Di Giorgio, P. Vierling, C. Ortiz Mellet, J. Defaye and J. M. García Fernández, Polycationic amphiphilic cyclodextrins as gene vectors: effect of the macrocyclic ring size on the DNA complexing and delivery properties, Org. Biomol. Chem., 2012, 10, 5570, 10.1039/c2ob25786f.
- V. Coviello, S. Sartini, L. Quattrini, C. Baraldi, M. C. Gamberini and C. La Motta, Cyclodextrin-based nanosponges for the targeted delivery of the anti-restenotic agent DB103: A novel opportunity for the local therapy of vessels wall subjected to percutaneous intervention, Eur. J. Pharm. Biopharm., 2017, 117, 276–285, DOI:10.1016/j.ejpb.2017.04.028.
- B. Luppi, F. Bigucci, G. Corace, A. Delucca, T. Cerchiara, M. Sorrenti, L. Catenacci, A. M. Di Pietra and V. Zecchi, Albumin nanoparticles carrying cyclodextrins for nasal delivery of the anti-Alzheimer drug tacrine, Eur. J. Pharm. Sci., 2011, 44, 559–565, DOI:10.1016/j.ejps.2011.10.002.
- D. S. Ory, E. A. Ottinger, N. Y. Farhat, K. A. King, X. Jiang, L. Weissfeld, E. Berry-Kravis, C. D. Davidson, S. Bianconi, L. A. Keener, R. Rao, A. Soldatos, R. Sidhu, K. A. Walters, X. Xu, A. Thurm, B. Solomon, W. J. Pavan, B. N. Machielse, M. Kao, S. A. Silber, J. C. McKew, C. C. Brewer, C. H. Vite, S. U. Walkley, C. P. Austin and F. D. Porter, Intrathecal 2-hydroxypropyl-β-cyclodextrin decreases neurological disease progression in Niemann-Pick disease, type C1: a non-randomised, open-label, phase 1–2 trial, Lancet, 2017, 390, 1758–1768, DOI:10.1016/S0140-6736(17)31465-4.
- L. Zhang, S. Yang, L. R. Wong, H. Xie and P. C.-L. Ho, In Vitro and In Vivo Comparison of Curcumin-Encapsulated Chitosan-Coated Poly(lactic-co-glycolic acid) Nanoparticles and Curcumin/Hydroxypropyl-β-Cyclodextrin Inclusion Complexes Administered Intranasally as Therapeutic Strategies for Alzheimer's Disease, Mol. Pharmaceutics, 2020, 17, 4256–4269, DOI:10.1021/acs.molpharmaceut.0c00675.
- Y. Khazaei Monfared, M. Mahmoudian, C. Cecone, F. Caldera, P. Zakeri-Milani, A. Matencio and F. Trotta, Stabilization and Anticancer Enhancing Activity of the Peptide Nisin by Cyclodextrin-Based Nanosponges against Colon and Breast Cancer Cells, Polymers, 2022, 14, 1–19, DOI:10.3390/polym14030594.
- T. Loftsson and D. Duchene, Cyclodextrins and their pharmaceutical applications, Int. J. Pharm., 2007, 329, 1–11, DOI:10.1016/j.ijpharm.2006.10.044.
- J. Szejtli, Introduction and General Overview of Cyclodextrin Chemistry, Chem. Rev., 1998, 98, 1743–1754, DOI:10.1021/cr970022c.
- W. Saenger, J. Jacob, K. Gessler, T. Steiner, D. Hoffmann, H. Sanbe, K. Koizumi, S. M. Smith and T. Takaha, Structures of the Common Cyclodextrins and Their Larger AnaloguesBeyond the Doughnut, Chem. Rev., 1998, 98, 1787–1802, DOI:10.1021/cr9700181.
- A. A. Sandilya, U. Natarajan and M. H. Priya, Molecular View into the Cyclodextrin Cavity: Structure and Hydration, ACS Omega, 2020, 5, 25655–25667, DOI:10.1021/acsomega.0c02760.
- G. Tiwari, R. Tiwari and A. Rai, Cyclodextrins in delivery systems: Applications, J. Pharm. BioAllied Sci., 2010, 2, 72, DOI:10.4103/0975-7406.67003.
- H. Arima, K. Yunomae, K. Miyake, T. Irie, F. Hirayama and K. Uekama, Comparative Studies of the Enhancing Effects of Cyclodextrins on the Solubility and Oral Bioavailability of Tacrolimus in Rats, J. Pharm. Sci., 2001, 90, 690–701, DOI:10.1002/jps.1025.
- P. Jansook, N. Ogawa and T. Loftsson, Cyclodextrins: structure, physicochemical properties and pharmaceutical applications, Int. J. Pharm., 2018, 535, 272–284, DOI:10.1016/j.ijpharm.2017.11.018.
-
J. L. Atwood, Comprehensive Supramolecular Chemistry II, Elsevier Ltd, 2017 Search PubMed.
- J. Li and X. Loh, Cyclodextrin-based supramolecular architectures: Syntheses, structures, and applications for drug and gene delivery, Adv. Drug Delivery Rev., 2008, 60, 1000–1017, DOI:10.1016/j.addr.2008.02.011.
- E. Bilensoy, O. Gürkaynak, A. L. Doğan and A. A. Hıncal, Safety and efficacy of amphiphilic β-cyclodextrin nanoparticles for paclitaxel delivery, Int. J. Pharm., 2008, 347, 163–170, DOI:10.1016/j.ijpharm.2007.06.051.
- V. Venuti, B. Rossi, A. Mele, L. Melone, C. Punta, D. Majolino, C. Masciovecchio, F. Caldera and F. Trotta, Tuning structural parameters for the optimization of drug delivery performance of cyclodextrin-based nanosponges, Expert Opin. Drug Delivery, 2017, 14, 331–340, DOI:10.1080/17425247.2016.1215301.
- X. Yao, J. Mu, L. Zeng, J. Lin, Z. Nie, X. Jiang and P. Huang, Stimuli-responsive cyclodextrin-based nanoplatforms for cancer treatment and theranostics, Mater. Horiz., 2019, 6, 846–870, 10.1039/C9MH00166B.
- H. Namazi and A. Heydari, Synthesis of β -cyclodextrin-based dendrimer as a novel encapsulation agent, Polym. Int., 2014, 63, 1447–1455, DOI:10.1002/pi.4637.
- R. Machín, J. R. Isasi and I. Vélaz, β-Cyclodextrin hydrogels as potential drug delivery systems, Carbohydr. Polym., 2012, 87, 2024–2030, DOI:10.1016/j.carbpol.2011.10.024.
- T. Yousef and N. Hassan, Supramolecular encapsulation of doxorubicin with β-cyclodextrin dendrimer: in vitro evaluation of controlled release and cytotoxicity, J. Inclusion Phenom. Macrocyclic Chem., 2017, 87, 105–115, DOI:10.1007/s10847-016-0682-4.
- M. Agüeros, V. Zabaleta, S. Espuelas, M. A. Campanero and J. M. Irache, Increased oral bioavailability of paclitaxel by its encapsulation through complex formation with cyclodextrins in poly(anhydride) nanoparticles, J. Controlled Release, 2010, 145, 2–8, DOI:10.1016/j.jconrel.2010.03.012.
- C. L. Gigliotti, R. Minelli, R. Cavalli, S. Occhipinti, G. Barrera, S. Pizzimenti, G. Cappellano, E. Boggio, L. Conti, R. Fantozzi, M. Giovarelli, F. Trotta, U. Dianzani and C. Dianzani, In Vitro and In Vivo Therapeutic Evaluation of Camptothecin-Encapsulated β-Cyclodextrin Nanosponges in Prostate Cancer, J. Biomed. Nanotechnol., 2016, 12, 114, DOI:10.1166/jbn.2016.2144.
- F. Trotta, F. Caldera, R. Cavalli, M. Soster, C. Riedo, M. Biasizzo, G. Uccello Barretta, F. Balzano and V. Brunella, Molecularly imprinted cyclodextrin nanosponges for the controlled delivery of L-DOPA: perspectives for the treatment of Parkinson's disease, Expert Opin. Drug Delivery, 2016, 13, 1671–1680, DOI:10.1080/17425247.2017.1248398.
- D. Lembo, S. Swaminathan, M. Donalisio, A. Civra, L. Pastero, D. Aquilano, P. Vavia, F. Trotta and R. Cavalli, Encapsulation of Acyclovir in new carboxylated cyclodextrin-based nanosponges improves the agent's antiviral efficacy, Int. J. Pharm., 2013, 443, 262–272, DOI:10.1016/j.ijpharm.2012.12.031.
- S. S. Dhule, P. Penfornis, T. Frazier, R. Walker, J. Feldman, G. Tan, J. He, A. Alb, V. John and R. Pochampally, Curcumin-loaded γ-cyclodextrin liposomal nanoparticles as delivery vehicles for osteosarcoma, Nanomed.: Nanotechnol. Biol. Med., 2012, 8, 440–451, DOI:10.1016/j.nano.2011.07.011.
- E. Soo, S. Thakur, Z. Qu, S. Jambhrunkar, H. S. Parekh and A. Popat, Enhancing delivery and cytotoxicity of resveratrol through a dual nanoencapsulation approach, J. Colloid Interface Sci., 2016, 462, 368–374, DOI:10.1016/j.jcis.2015.10.022.
- Y. Li, Z.-D. He, Q.-E. Zheng, C. Hu and W.-F. Lai, Hydroxypropyl-β-cyclodextrin for Delivery of Baicalin via Inclusion Complexation by Supercritical Fluid Encapsulation, Molecules, 2018, 23, 1169, DOI:10.3390/molecules23051169.
- D. Desai and P. Shende, Monodispersed cyclodextrin-based nanocomplex of neuropeptide Y for targeting MCF-7 cells using a central composite design, J. Drug Delivery Sci. Technol., 2021, 65, 102692, DOI:10.1016/j.jddst.2021.102692.
- Z. Li, H. Yin, Z. Zhang, K. L. Liu and J. Li, Supramolecular Anchoring of DNA Polyplexes in Cyclodextrin-Based Polypseudorotaxane Hydrogels for Sustained Gene Delivery, Biomacromolecules, 2012, 13, 3162–3172, DOI:10.1021/bm300936x.
- J. H. Choi, A. Park, W. Lee, J. Youn, M. A. Rim, W. Kim, N. Kim, J. E. Song and G. Khang, Preparation and characterization of an injectable dexamethasone-cyclodextrin complexes-loaded gellan gum hydrogel for cartilage tissue engineering, J. Controlled Release, 2020, 327, 747–765, DOI:10.1016/j.jconrel.2020.08.049.
- D. H. Schwarz, A. Engelke and G. Wenz, Solubilizing steroidal drugs by β-cyclodextrin derivatives, Int. J. Pharm., 2017, 531, 559–567, DOI:10.1016/j.ijpharm.2017.07.046.
- R. Pushpalatha, S. Selvamuthukumar and D. Kilimozhi, Cyclodextrin nanosponge based hydrogel for the transdermal co-delivery of curcumin and resveratrol: Development, optimization, in vitro and ex vivo evaluation, J. Drug Delivery Sci. Technol., 2019, 52, 55–64, DOI:10.1016/j.jddst.2019.04.025.
- D. Desai and P. Shende, Cyclodextrin-based gefitinib nanobubbles for synergistic apoptosis in lung cancer, Mater. Technol., 2021, 1–12, DOI:10.1080/10667857.2021.1969493.
- P. J. Salústio, P. Pontes, C. Conduto, I. Sanches, C. Carvalho, J. Arrais and H. M. C. Marques, Advanced Technologies for Oral Controlled Release: Cyclodextrins for Oral Controlled Release, AAPS PharmSciTech, 2011, 12, 1276–1292, DOI:10.1208/s12249-011-9690-2.
- M. Liu, Y. Zheng, C. Wang, J. Xie, B. Wang, Z. Wang, J. Han, D. Sun and M. Niu, Improved stability of (+)-catechin and (−)-epicatechin by complexing with hydroxypropyl-β-cyclodextrin: Effect of pH, temperature and configuration, Food Chem., 2016, 196, 148–154, DOI:10.1016/j.foodchem.2015.09.016.
- F. Leroy-Lechat, D. Wouessidjewe, J.-P. Andreux, F. Puisieux and D. Duchêne, Evaluation of the cytotoxicity of cyclodextrins and hydroxypropylated derivatives, Int. J. Pharm., 1994, 101, 97–103, DOI:10.1016/0378-5173(94)90080-9.
- E. Róka, Z. Ujhelyi, M. Deli, A. Bocsik, É. Fenyvesi, L. Szente, F. Fenyvesi, M. Vecsernyés, J. Váradi, P. Fehér, R. Gesztelyi, C. Félix, F. Perret and I. Bácskay, Evaluation of the Cytotoxicity of α-Cyclodextrin Derivatives on the Caco-2 Cell Line and Human Erythrocytes, Molecules, 2015, 20, 20269–20285, DOI:10.3390/molecules201119694.
- S. Shityakov, I. Puskás, K. Pápai, E. Salvador, N. Roewer, C. Förster and J.-A. Broscheit, Sevoflurane-Sulfobutylether-β-Cyclodextrin Complex: Preparation, Characterization, Cellular Toxicity, Molecular Modeling and Blood-Brain Barrier Transport Studies, Molecules, 2015, 20, 10264–10279, DOI:10.3390/molecules200610264.
- L. Matilainen, T. Toropainen, H. Vihola, J. Hirvonen, T. Järvinen, P. Jarho and K. Järvinen, In vitro toxicity and permeation of cyclodextrins in Calu-3 cells, J. Controlled Release, 2008, 126, 10–16, DOI:10.1016/j.jconrel.2007.11.003.
- S. M. N. Simões, A. Rey-Rico, A. Concheiro and C. Alvarez-Lorenzo, Supramolecular cyclodextrin-based drug nanocarriers, Chem. Commun., 2015, 51, 6275–6289, 10.1039/C4CC10388B.
- T. Irie and K. Uekama, Pharmaceutical Applications of Cyclodextrins. III. Toxicological Issues and Safety Evaluation, J. Pharm. Sci., 1997, 86, 147–162, DOI:10.1021/js960213f.
- S. Desai, A. Poddar and K. Sawant, Formulation of cyclodextrin inclusion complex-based orally disintegrating tablet of eslicarbazepine acetate for improved oral bioavailability, Mater. Sci. Eng., C, 2016, 58, 826–834, DOI:10.1016/j.msec.2015.09.019.
- M. S. Al-Dosari and X. Gao, Nonviral Gene Delivery: Principle, Limitations, and Recent Progress, AAPS J., 2009, 11, 671, DOI:10.1208/s12248-009-9143-y.
- K. A. Whitehead, J. E. Dahlman, R. S. Langer and D. G. Anderson, Silencing or Stimulation? siRNA Delivery and the Immune System, Annu. Rev. Chem. Biomol. Eng., 2011, 2, 77–96, DOI:10.1146/annurev-chembioeng-061010-114133.
- N. Khairuddin, M. P. Gantier, S. J. Blake, S. Y. Wu, M. A. Behlke, B. R. Williams and N. A. McMillan, siRNA-induced immunostimulation through TLR7 promotes antitumoral activity against HPV-driven tumors in vivo, Immunol. Cell Biol., 2012, 90, 187–196, DOI:10.1038/icb.2011.19.
- J. Villemejane and L. M. Mir, Physical methods of nucleic acid transfer: general concepts and applications, Br. J. Pharmacol., 2009, 157, 207–219, DOI:10.1111/j.1476-5381.2009.00032.x.
- L. K. Medina-Kauwe, J. Xie and S. Hamm-Alvarez, Intracellular trafficking of nonviral vectors, Gene Ther., 2005, 12, 1734–1751, DOI:10.1038/sj.gt.3302592.
- G. L. Lukacs, P. Haggie, O. Seksek, D. Lechardeur, N. Freedman and A. S. Verkman, Size-dependent DNA Mobility in Cytoplasm and Nucleus, J. Biol. Chem., 2000, 275, 1625–1629, DOI:10.1074/jbc.275.3.1625.
- R. Bastos, N. Panté and B. Burke, Nuclear Pore Complex Proteins, Int. Rev. Cytol., 1996, 257–302, DOI:10.1016/S0074-7696(08)62619-4.
- T. Ura, K. Okuda and M. Shimada, Developments in Viral Vector-Based Vaccines, Vaccines, 2014, 2, 624–641, DOI:10.3390/vaccines2030624.
- S. Patil, Y. G. Gao, X. Lin, Y. Li, K. Dang, Y. Tian, W. J. Zhang, S. F. Jiang, A. Qadir and A. R. Qian, The Development of Functional Non-Viral Vectors for Gene Delivery, Int. J. Mol. Sci., 2019, 20, 5491, DOI:10.3390/ijms20215491.
- H. Zu and D. Gao, Non-viral Vectors in Gene Therapy: Recent Development, Challenges, and Prospects, AAPS J., 2021, 23, 78, DOI:10.1208/s12248-021-00608-7.
- H. Elsana, T. O. B. Olusanya, J. Carr-Wilkinson, S. Darby, A. Faheem and A. A. Elkordy, Evaluation of novel cationic gene based liposomes with cyclodextrin prepared by thin film hydration and microfluidic systems, Sci. Rep., 2019, 9, 15120, DOI:10.1038/s41598-019-51065-4.
- L. S. Devi, C. Casadidio, M. R. Gigliobianco, P. Di Martino and R. Censi, Multifunctionality of cyclodextrin-based polymeric nanoparticulate delivery systems for chemotherapeutics, combination therapy, and theranostics, Int. J. Pharm., 2024, 654, 123976, DOI:10.1016/j.ijpharm.2024.123976.
- H. Huang, H. Yu, G. Tang, Q. Wang and J. Li, Low molecular weight polyethylenimine cross-linked by 2-hydroxypropyl-γ-cyclodextrin coupled to peptide targeting HER2 as a gene delivery vector, Biomaterials, 2010, 31, 1830–1838, DOI:10.1016/j.biomaterials.2009.11.012.
- H. Arima, S. Yamashita, Y. Mori, Y. Hayashi, K. Motoyama, K. Hattori, T. Takeuchi, H. Jono, Y. Ando and F. Hirayama, In Vitro and In Vivo gene delivery mediated by Lactosylated Dendrimer/α-Cyclodextrin Conjugates (G2) into Hepatocytes, J. Controlled Release, 2010, 146, 106–117, DOI:10.1016/j.jconrel.2010.05.030.
- A. Kargaard, J. P. G. Sluijter and B. Klumperman, Polymeric siRNA gene delivery – transfection efficiency versus cytotoxicity, J. Controlled Release, 2019, 316, 263–291, DOI:10.1016/j.jconrel.2019.10.046.
- M. Wojnilowicz, A. Glab, A. Bertucci, F. Caruso and F. Cavalieri, Super-resolution Imaging of Proton Sponge-Triggered Rupture of Endosomes and Cytosolic Release of Small Interfering RNA, ACS Nano, 2019, 13, 187–202, DOI:10.1021/acsnano.8b05151.
- R. Faria, Â. Sousa, A. R. Neves, J. A. Queiroz and D. Costa, Methotrexate-plasmid DNA polyplexes for cancer therapy: Characterization, cancer cell targeting ability and tuned in vitro transfection, J. Mol. Liq., 2019, 292, 111391, DOI:10.1016/j.molliq.2019.111391.
- M. B. Parmar, R. B. KC, R. Lobenberg and H. Uludag, Additive Polyplexes to Undertake siRNA Therapy against CDC20 and Survivin in Breast Cancer Cells, Biomacromolecules, 2018, 19, 4193–4206, DOI:10.1021/acs.biomac.8b00918.
- H. Lv, S. Zhang, B. Wang, S. Cui and J. Yan, Toxicity of cationic lipids and cationic polymers in gene delivery, J. Controlled Release, 2006, 114, 100–109, DOI:10.1016/j.jconrel.2006.04.014.
- R. M. Haley, R. Gottardi, R. Langer and M. J. Mitchell, Cyclodextrins in drug delivery: applications in gene and combination therapy, Drug Delivery Transl. Res., 2020, 10, 661–677, DOI:10.1007/s13346-020-00724-5.
- H. Gonzalez, S. J. Hwang and M. E. Davis, New Class of Polymers for the Delivery of Macromolecular Therapeutics, Bioconjugate Chem., 1999, 10, 1068–1074, DOI:10.1021/bc990072j.
- M. L. Patil, M. Zhang and T. Minko, Multifunctional Triblock Nanocarrier (PAMAM-PEG-PLL) for the Efficient Intracellular siRNA Delivery and Gene Silencing, ACS Nano, 2011, 5, 1877–1887, DOI:10.1021/nn102711d.
- L. Albertazzi, L. Gherardini, M. Brondi, S. Sulis Sato, A. Bifone, T. Pizzorusso, G. M. Ratto and G. Bardi, In Vivo Distribution and Toxicity of PAMAM Dendrimers in the Central Nervous System Depend on Their Surface Chemistry, Mol. Pharmaceutics, 2013, 10, 249–260, DOI:10.1021/mp300391v.
- S. P. Mukherjee, M. Davoren and H. J. Byrne, In vitro mammalian cytotoxicological study of PAMAM dendrimers – towards quantitative structure activity relationships, Toxicol. In Vitro, 2010, 24, 169–177, DOI:10.1016/j.tiv.2009.09.014.
- R. Araújo, S. Santos, E. Igne Ferreira and J. Giarolla, New Advances in General Biomedical Applications of PAMAM Dendrimers, Molecules, 2018, 23, 2849, DOI:10.3390/molecules23112849.
- A. Janaszewska, J. Lazniewska, P. Trzepiński, M. Marcinkowska and B. Klajnert-Maculewicz, Cytotoxicity of Dendrimers, Biomolecules, 2019, 9, 330, DOI:10.3390/biom9080330.
- G. Jiang, S.-H. Min, M.-N. Kim, D.-C. Lee, M.-J. Lim and Y.-I. Yeom, Alginate/PEI/DNA polyplexes: a new gene delivery system, Acta Pharm. Sin. B, 2006, 41, 439 CAS.
- P. Chollet, M. C. Favrot, A. Hurbin and J.-L. Coll, Side-effects of a systemic injection of linear polyethylenimine-DNA complexes, J. Gene Med., 2002, 4, 84–91, DOI:10.1002/jgm.237.
- P. Lv, C. Zhou, Y. Zhao, X. Liao and B. Yang, Modified-epsilon-polylysine-grafted-PEI-β-cyclodextrin supramolecular carrier for gene delivery, Carbohydr. Polym., 2017, 168, 103–111, DOI:10.1016/j.carbpol.2017.02.036.
- C. Englert, M. Fevre, R. J. Wojtecki, W. Cheng, Q. Xu, C. Yang, X. Ke, M. Hartlieb, K. Kempe, J. M. García, R. J. Ono, U. S. Schubert, Y. Y. Yang and J. L. Hedrick, Facile carbohydrate-mimetic modifications of poly(ethylene imine) carriers for gene delivery applications, Polym. Chem., 2016, 7, 5862–5872, 10.1039/C6PY00940A.
- M. R. Donthi, S. R. Munnangi, K. V. Krishna, S. A. Marathe, R. N. Saha, G. Singhvi and S. K. Dubey, Formulating Ternary Inclusion Complex of Sorafenib Tosylate Using β-Cyclodextrin and Hydrophilic Polymers: Physicochemical Characterization and In Vitro Assessment, AAPS PharmSciTech, 2022, 23, 254, DOI:10.1208/s12249-022-02406-6.
- V. Oliveri, F. Bellia, M. Viale, I. Maric and G. Vecchio, Linear polymers of β and γ cyclodextrins with a polyglutamic acid backbone as carriers for doxorubicin, Carbohydr. Polym., 2017, 177, 355–360, DOI:10.1016/j.carbpol.2017.08.103.
- G. Chen and M. Jiang, Cyclodextrin-based inclusion complexation bridging supramolecular chemistry and macromolecular self-assembly, Chem. Soc. Rev., 2011, 40, 2254, 10.1039/c0cs00153h.
- Y.-H. Zhang, Y. Chen, Y.-M. Zhang, Y. Yang, J.-T. Chen and Y. Liu, Recycling Gene Carrier with High Efficiency and Low Toxicity Mediated by L-Cystine-Bridged Bis(β-cyclodextrin)s, Sci. Rep., 2015, 4, 7471, DOI:10.1038/srep07471.
- C. Xu, B. Yu, Y. Qi, N. Zhao and F. Xu, Versatile Types of Cyclodextrin-Based Nucleic Acid Delivery Systems, Adv. Healthcare Mater., 2021, 10, 2001183, DOI:10.1002/adhm.202001183.
- C. Facciotti, V. Saggiomo, S. van Hurne, A. Bunschoten, R. Kaup and A. H. Velders, Oxidant-responsive ferrocene-based cyclodextrin complex coacervate core micelles, Supramol. Chem., 2020, 32, 30–38, DOI:10.1080/10610278.2019.1685094.
- L. Yin-Ku, W. Shiu-Wei and L. Ren-Shen, Photo and redox dual-stimuli-responsive β-cyclodextrin-ferrocene supramolecules for drug delivery, J. Macromol. Sci., Part A: Pure Appl. Chem., 2021, 58, 8–21, DOI:10.1080/10601325.2020.1814158.
- B. Jiang, H. Guo, L. Zhao, B. Xu, C. Wang, C. Liu and H. Fan, Fabrication of a β-cyclodextrin-based self-assembly containing a redox-responsive ferrocene, Soft Matter, 2020, 16, 125–131, 10.1039/C9SM02049G.
- H. Guo, B. Jiang, J. Zhou, L. Zhao, B. Xu and C. Liu, Self-assembly of β-cyclodextrin-derived amphiphile with a photo responsive guest, Colloids Surf., A, 2019, 579, 123683, DOI:10.1016/j.colsurfa.2019.123683.
- J. Zhang, Z. H. Zhou, L. Li, Y. L. Luo, F. Xu and Y. Chen, Dual Stimuli-Responsive Supramolecular Self-Assemblies Based on the Host-Guest Interaction between β-Cyclodextrin and Azobenzene for Cellular Drug Release, Mol. Pharmaceutics, 2020, 17, 1100–1113, DOI:10.1021/acs.molpharmaceut.9b01142.
- G. Wenz, B.-H. Han and A. Müller, Cyclodextrin rotaxanes and polyrotaxanes, Chem. Rev., 2006, 106, 782–817, DOI:10.1021/cr970027.
- A. Yamashita, H. S. Choi, T. Ooya, N. Yui, H. Akita, K. Kogure and H. Harashima, Improved Cell Viability of Linear Polyethylenimine through γ-Cyclodextrin Inclusion for Effective Gene Delivery, ChemBioChem, 2006, 7, 297–302, DOI:10.1002/cbic.200500348.
-
N. Yui, R. Katoono and A. Yamashita, in Functional Cyclodextrin Polyrotaxanes for Drug Delivery, Inclusion Polymers, 2009, pp. 115–173 Search PubMed.
- C. Xu, Y.-L. Wu, Z. Li and X. J. Loh, Cyclodextrin-based sustained gene release systems: a supramolecular solution towards clinical applications, Mater. Chem. Front., 2019, 3, 181–192, 10.1039/C8QM00570B.
- A.-K. Minnaert, G. R. Dakwar, J. M. Benito, J. M. García Fernández, W. Ceelen, S. C. De Smedt and K. Remaut, High-Pressure Nebulization as Application Route for the Peritoneal Administration of siRNA Complexes, Macromol. Biosci., 2017, 17, 1700024, DOI:10.1002/mabi.201700024.
- M. Malhotra, M. Gooding, J. C. Evans, D. O’Driscoll, R. Darcy and C. M. O’Driscoll, Cyclodextrin-siRNA conjugates as versatile gene silencing agents, Eur. J. Pharm. Sci., 2018, 114, 30–37, DOI:10.1016/j.ejps.2017.11.024.
- D. Manzanares, M. D. Pérez-Carrión, J. L. Jiménez Blanco, C. Ortiz Mellet, J. M. García Fernández and V. Ceña, Cyclodextrin-Based Nanostructure Efficiently Delivers siRNA to Glioblastoma Cells Preferentially via Macropinocytosis, Int. J. Mol. Sci., 2020, 21, 9306, DOI:10.3390/ijms21239306.
- Y. Zhang, L. Yu, J. Zhu and R. Gong, Preparation of folate and carboxymethyl-β-cyclodextrin grafted trimethyl chitosan nanoparticles as co-carrier of doxorubicin and siRNA, React. Funct. Polym., 2021, 161, 104867, DOI:10.1016/j.reactfunctpolym.2021.104867.
- J. C. Evans, M. Malhotra, K. Sweeney, R. Darcy, C. C. Nelson, B. G. Hollier and C. M. O’Driscoll, Folate-targeted amphiphilic cyclodextrin nanoparticles incorporating a fusogenic peptide deliver therapeutic siRNA and inhibit the invasive capacity of 3D prostate cancer tumours, Int. J. Pharm., 2017, 532, 511–518, DOI:10.1016/j.ijpharm.2017.09.013.
- K. A. Fitzgerald, M. Malhotra, M. Gooding, F. Sallas, J. C. Evans, R. Darcy and C. M. O’Driscoll, A novel, anisamide-targeted cyclodextrin nanoformulation for siRNA delivery to prostate cancer cells expressing the Sigma-1 receptor, Int. J. Pharm., 2016, 499, 131–145, DOI:10.1016/j.ijpharm.2015.12.055.
- J. C. Evans, M. Malhotra, J. Guo, J. P. O’Shea, K. Hanrahan, A. O’Neill, W. D. Landry, B. T. Griffin, R. Darcy, R. W. Watson and C. M. O’Driscoll, Folate-targeted amphiphilic cyclodextrin.siRNA nanoparticles for prostate cancer therapy exhibit PSMA mediated uptake, therapeutic gene silencing in vitro and prolonged circulation in vivo, Nanomed.: Nanotechnol. Biol. Med., 2016, 12, 2341–2351, DOI:10.1016/j.nano.2016.06.014.
- M. Gooding, M. Malhotra, D. J. McCarthy, B. M. D. C. Godinho, J. F. Cryan, R. Darcy and C. M. O’Driscoll, Synthesis and characterization of rabies virus glycoprotein-tagged amphiphilic cyclodextrins for siRNA delivery in human glioblastoma cells: In vitro analysis, Eur. J. Pharm. Sci., 2015, 71, 80–92, DOI:10.1016/j.ejps.2015.02.007.
- X. Chen, T. Chen, L. Zhang, Z. Wang, Q. Zhou, T. Huang, C. Ge, H. Xu, M. Zhu, F. Zhao, M. Yao, H. Tian, H. Li, X. Zhu and J. Li, Cyclodextrin-mediated formation of porous RNA nanospheres and their application in synergistic targeted therapeutics of hepatocellular carcinoma, Biomaterials, 2020, 261, 120304, DOI:10.1016/j.biomaterials.2020.120304.
- F.-Q. Li, Q.-L. Yu, Y.-H. Liu, H.-J. Yu, Y. Chen and Y. Liu, Highly efficient photocontrolled targeted delivery of siRNA by a cyclodextrin-based supramolecular nanoassembly, Chem. Commun., 2020, 56, 3907–3910, 10.1039/D0CC00629G.
- J. Zhang, N. Jing, X. Fan and X. Tang, Photoregulation of Gene Expression with Amantadine-Modified Caged siRNAs through Host–Guest Interactions, Chem. – Eur. J., 2020, 26, 14002–14010, DOI:10.1002/chem.202003084.
- D. Wang, T. Wang, Z. Xu, H. Yu, B. Feng, J. Zhang, C. Guo, Q. Yin, Z. Zhang and Y. Li, Cooperative Treatment of Metastatic Breast Cancer Using Host-Guest Nanoplatform Coloaded with Docetaxel and siRNA, Small, 2016, 12, 488–498, DOI:10.1002/smll.201502913.
- Q. Xiong, M. Cui, Y. Bai, Y. Liu, D. Liu and T. Song, A supramolecular nanoparticle system based on β-cyclodextrin-conjugated poly-l-lysine and hyaluronic acid for co-delivery of gene and chemotherapy agent targeting hepatocellular carcinoma, Colloids Surf., B, 2017, 155, 93–103, DOI:10.1016/j.colsurfb.2017.04.008.
- Q. Xiong, Y. Bai, R. Shi, J. Wang, W. Xu, M. Zhang and T. Song, Preferentially released miR-122 from cyclodextrin-based star copolymer nanoparticle enhances hepatoma chemotherapy by apoptosis induction and cytotoxics efflux inhibition, Bioact. Mater., 2021, 6, 3744–3755, DOI:10.1016/j.bioactmat.2021.03.026.
- H. Arima, F. Kihara, F. Hirayama and K. Uekama, Enhancement of Gene Expression by Polyamidoamine Dendrimer Conjugates with α-, β-, and γ-Cyclodextrins, Bioconjugate Chem., 2001, 12, 476–484, DOI:10.1021/bc000111n.
- J. Qiu, L. Kong, X. Cao, A. Li, P. Wei, L. Wang, S. Mignani, A.-M. Caminade, J.-P. Majoral and X. Shi, Enhanced Delivery of Therapeutic siRNA into Glioblastoma Cells Using Dendrimer-Entrapped Gold Nanoparticles Conjugated with β-Cyclodextrin, Nanomaterials, 2018, 8, 131, DOI:10.3390/nano8030131.
- Y. Hayashi, T. Higashi, K. Motoyama, H. Jono, Y. Ando, R. Onodera and H. Arima, Hepatocyte-targeted delivery of siRNA polyplex with PEG-modified lactosylated dendrimer/cyclodextrin conjugates for transthyretin-related amyloidosis therapy, Biol. Pharm. Bull., 2019, 42, 1679–1688, DOI:10.1248/bpb.b19-00278.
- T. Anno, T. Higashi, K. Motoyama, F. Hirayama, K. Uekama and H. Arima, Possible enhancing mechanisms for gene transfer activity of glucuronylglucosyl-β-cyclodextrin/dendrimer conjugate, Int. J. Pharm., 2012, 426, 239–247, DOI:10.1016/j.ijpharm.2012.01.039.
- A. F. Abdelwahab, A. Ohyama, T. Higashi, K. Motoyama, K. A. Khaled, H. A. Sarhan, A. K. Hussein and H. Arima, Preparation and evaluation of polyamidoamine dendrimer conjugate with glucuronylglucosyl- β -cyclodextrin (G3) as a novel carrier for siRNA, J. Drug Targeting, 2014, 22, 927–934, DOI:10.3109/1061186X.2014.950663.
- A. F. A. Mohammed, T. Higashi, K. Motoyama, A. Ohyama, R. Onodera, K. A. Khaled, H. A. Sarhan, A. K. Hussein and H. Arima, In Vitro and In Vivo Co-delivery of siRNA and Doxorubicin by Folate-PEG-Appended Dendrimer/Glucuronylglucosyl-β-Cyclodextrin Conjugate, AAPS J., 2019, 21, 54, DOI:10.1208/s12248-019-0327-9.
- S. B. Ghodke, J. N. Parkar, A. R. Deshpande, P. P. Dandekar and R. D. Jain, Structure–Activity Relationship of Polyester-Based Cationic Polyrotaxane Vector-Mediated In Vitro siRNA Delivery: Effect on Gene Silencing Efficiency, ACS Appl. Bio Mater., 2020, 3, 7500–7514, DOI:10.1021/acsabm.0c00717.
- H. Elsana, S. Mysina, E. A. Elkordy, J. Carr-Wilkinson and A. A. Elkordy, Insights into the Influences of Carboxymethyl-β-Cyclodextrin on DNA Formulations Characteristics and Gene Transfection Efficiency, Curr. Drug Delivery, 2018, 15, 867–878, DOI:10.2174/1567201815666180226115503.
- A. Štimac, M. Tokić, A. Ljubetič, T. Vuletić, M. Šekutor, J. Požar, K. Leko, M. Hanževački, L. Frkanec and R. Frkanec, Functional self-assembled nanovesicles based on β-cyclodextrin, liposomes and adamantyl guanidines as potential nonviral gene delivery vectors, Org. Biomol. Chem., 2019, 17, 4640–4651, 10.1039/C9OB00488B.
- Y. Zhang, Q. Jiang, M. Wojnilowicz, S. Pan, Y. Ju, W. Zhang, J. Liu, R. Zhuo and X. Jiang, Acid-sensitive poly(β-cyclodextrin)-based multifunctional supramolecular gene vector, Polym. Chem., 2018, 9, 450–462, 10.1039/c7py01847a.
- J. Wang, L. Liu, J. Chen, M. Deng, X. Feng and L. Chen, Supramolecular nanoplatforms via cyclodextrin host-guest recognition for synergistic gene-photodynamic therapy, Eur. Polym. J., 2019, 118, 222–230, DOI:10.1016/j.eurpolymj.2019.04.051.
- S. Sahoo, S. Bera and D. Dhara, Histidine-Based Reduction-Sensitive Star-Polymer Inclusion Complex as a Potential DNA Carrier: Biophysical Studies Using Time-Resolved Fluorescence as an Important Tool, Langmuir, 2020, 36, 11262–11273, DOI:10.1021/acs.langmuir.0c01636.
- H. Li, E. Peng, F. Zhao, J. Li and J. Xue, Supramolecular Surface Functionalization of Iron Oxide Nanoparticles with α-Cyclodextrin-Based Cationic Star Polymer for Magnetically-Enhanced Gene Delivery, Pharmaceutics, 2021, 13, 1884, DOI:10.3390/pharmaceutics13111884.
- L. Ke, Z. Li, X. Fan, X. J. Loh, H. Cheng, Y. Wu and Z. Li, Cyclodextrin-Based Hybrid Polymeric Complex to Overcome Dual Drug Resistance Mechanisms for Cancer Therapy, Polymers, 2021, 13, 1254, DOI:10.3390/polym13081254.
- Q. Jiang, S. Guan, Y. Zhang, Y. Sun and X. Jiang, Targeted and fluorescence traceable multifunctional host-guest supramolecular gene delivery platform based on poly(cyclodextrin) and rhodamine conjugated disulfide-containing azobenzene-terminated branched polymer, Int. J. Polym. Mater. Polym. Biomater., 2022, 1–13, DOI:10.1080/00914037.2022.2029438.
- Y. Jiang, X. Pan, J. Chang, W. Niu, W. Hou, H. Kuai, Z. Zhao, J. Liu, M. Wang and W. Tan, Supramolecularly Engineered Circular Bivalent Aptamer for Enhanced Functional Protein Delivery, J. Am. Chem. Soc., 2018, 140, 6780–6784, DOI:10.1021/jacs.8b03442.
- Y. Shen, M. Li, T. Liu, J. Liu, Y. Xie, J. Zhang, S. Xu and H. Liu, A dual-functional HER2 aptamer-conjugated, pH-activated mesoporous silica nanocarrier-based drug delivery system provides in vitro synergistic cytotoxicity in HER2-positive breast cancer cells, Int. J. Nanomed., 2019, 14, 4029–4044, DOI:10.2147/IJN.S201688.
- X. Li, Y. Yu, Q. Ji and L. Qiu, Targeted delivery of anticancer drugs by aptamer AS1411 mediated Pluronic F127/cyclodextrin-linked polymer composite micelles, Nanomedicine, 2015, 11, 175–184, DOI:10.1016/j.nano.2014.08.013.
- M. Hasanzadeh, N. Razmi, A. Mokhtarzadeh, N. Shadjou and S. Mahboob, Aptamer based assay of plated-derived grow factor in unprocessed human plasma sample and MCF-7 breast cancer cell lysates using gold nanoparticle supported α-cyclodextrin, Int. J. Biol. Macromol., 2018, 108, 69–80, DOI:10.1016/j.ijbiomac.2017.11.149.
- Y. Wu, H. Bi, G. Ning, Z. Xu, G. Liu, Y. Wang and Y. Zhao, Cyclodextrin subject-object recognition-based aptamer sensor for sensitive and selective detection of tetracycline, J. Solid State Electrochem., 2020, 24, 2365–2372, DOI:10.1007/s10008-020-04751-7.
- H. He, C. Xie, L. Yao, G. Ning and Y. Wang, A Sensitive Fluorescent Assay for Tetracycline Detection Based on Triple-helix Aptamer Probe and Cyclodextrin Supramolecular Inclusion, J. Fluoresc., 2021, 31, 63–71, DOI:10.1007/s10895-020-02631-x.
- G. Zu, Y. Cao, J. Dong, Q. Zhou, P. van Rijn, M. Liu and R. Pei, Development of an Aptamer-Conjugated Polyrotaxane-Based Biodegradable Magnetic Resonance Contrast Agent for Tumor-Targeted Imaging, ACS Appl. Bio Mater., 2019, 2, 406–416, DOI:10.1021/acsabm.8b00639.
- K. H. Wong, Y. Xie, X. Huang, K. Kadota, X.-S. Yao, Y. Yu, X. Chen, A. Lu and Z. Yang, Delivering Crocetin across the Blood-Brain Barrier by Using γ-Cyclodextrin to Treat Alzheimer's Disease, Sci. Rep., 2020, 10, 3654, DOI:10.1038/s41598-020-60293-y.
- R. Yokoyama, T. Taharabaru, T. Nishida, Y. Ohno, Y. Maeda, M. Sato, K. Ishikura, K. Yanagihara, H. Takagi, T. Nakamura, S. Ito, S. Ohtsuki, H. Arima, R. Onodera, T. Higashi and K. Motoyama, Lactose-appended β-cyclodextrin as an effective nanocarrier for brain delivery, J. Controlled Release, 2020, 328, 722–735, DOI:10.1016/j.jconrel.2020.09.043.
- S. Shityakov, R. E. Salmas, S. Durdagi, E. Salvador, K. Pápai, M. J. Yáñez-Gascón, H. Pérez-Sánchez, I. Puskás, N. Roewer, C. Förster and J.-A. Broscheit, Characterization, in Vivo Evaluation, and Molecular Modeling of Different Propofol–Cyclodextrin Complexes To Assess Their Drug Delivery Potential at the Blood–Brain Barrier Level, J. Chem. Inf. Model., 2016, 56, 1914–1922, DOI:10.1021/acs.jcim.6b00215.
- A. M. O’Mahony, B. M. D. C. Godinho, J. Ogier, M. Devocelle, R. Darcy, J. F. Cryan and C. M. O’Driscoll, Click-Modified Cyclodextrins as Nonviral Vectors for Neuronal siRNA Delivery, ACS Chem. Neurosci., 2012, 3, 744–752, DOI:10.1021/cn3000372.
- A. M. O’Mahony, S. Desgranges, J. Ogier, A. Quinlan, M. Devocelle, R. Darcy, J. F. Cryan and C. M. O’Driscoll, In Vitro Investigations of the Efficacy of Cyclodextrin-siRNA Complexes Modified with Lipid-PEG-Octaarginine: Towards a Formulation Strategy for Non-viral Neuronal siRNA Delivery, Pharm. Res., 2013, 30, 1086–1098, DOI:10.1007/s11095-012-0945-8.
- A. M. O’Mahony, J. Ogier, R. Darcy, J. F. Cryan and C. M. O’Driscoll, Cationic and PEGylated Amphiphilic Cyclodextrins: Co-Formulation Opportunities for Neuronal Sirna Delivery, PLoS One, 2013, 8, e66413, DOI:10.1371/journal.pone.0066413.
- A. Díaz-Moscoso, N. Guilloteau, C. Bienvenu, A. Méndez-Ardoy, J. L. Jiménez Blanco, J. M. Benito, L. Le Gourriérec, C. Di Giorgio, P. Vierling, J. Defaye, C. Ortiz Mellet and J. M. García Fernández, Mannosyl-coated nanocomplexes from amphiphilic cyclodextrins and pDNA for site-specific gene delivery, Biomaterials, 2011, 32, 7263–7273, DOI:10.1016/j.biomaterials.2011.06.025.
- A. Méndez-Ardoy, A. Díaz-Moscoso, C. Ortiz Mellet, C. Di Giorgio, P. Vierling, J. M. Benito and J. M. García Fernández, Harmonized tuning of nucleic acid and lectin binding properties with multivalent cyclodextrins for macrophage-selective gene delivery, RSC Adv., 2015, 5, 76464–76471, 10.1039/C5RA16087A.
- J. Guo, J. R. Ogier, S. Desgranges, R. Darcy and C. O′Driscoll, Anisamide-targeted cyclodextrin nanoparticles for siRNA delivery to prostate tumours in mice, Biomaterials, 2012, 33, 7775–7784, DOI:10.1016/j.biomaterials.2012.07.012.
- Z. Zhang, T. Wan, Y. Chen, Y. Chen, H. Sun, T. Cao, Z. Songyang, G. Tang, C. Wu, Y. Ping, F.-J. Xu and J. Huang, Cationic Polymer-Mediated CRISPR/Cas9 Plasmid Delivery for Genome Editing, Macromol. Rapid Commun., 2019, 40, 1800068, DOI:10.1002/marc.201800068.
- H. Chen, H. Jia, H. P. Tham, Q. Qu, P. Xing, J. Zhao, S. Z. F. Phua, G. Chen and Y. Zhao, Theranostic Prodrug Vesicles for Imaging Guided Codelivery of Camptothecin and siRNA in Synergetic Cancer Therapy, ACS Appl. Mater. Interfaces, 2017, 9, 23536–23543, DOI:10.1021/acsami.7b06936.
- A. Palaniappan, X. Li, F. E. Tay, J. Li and X. Su, Cyclodextrin functionalized mesoporous silica films on quartz crystal microbalance for enhanced gas sensing, Sens. Actuators, B, 2006, 119, 220–226, DOI:10.1016/j.snb.2005.12.015.
- J. Li, X. Jin, M. Feng, S. Huang and J. Feng, Ultrasensitive and highly selective electrochemical biosensor for HIV gene detection based on amino-reduced graphene oxide and β-cyclodextrin modified glassy carbon electrode, Int. J. Electrochem. Sci., 2020, 15, 2727–2738 CrossRef CAS.
- Y. Huang, J. Ji, J. Zhang, F. Wang and J. Lei, Host–guest recognition-regulated aggregation-induced emission for in situ imaging of MUC1 protein, Chem. Commun., 2020, 56, 313–316, 10.1039/C9CC07697B.
-
M. A. Sayeed, S. P. Mohanty, E. Kougianos and H. P. Zaveri, An IoT-based Drug Delivery System for Refractory Epilepsy, in: 2019 IEEE International Conference on Consumer Electronics (ICCE), IEEE, 2019: 1–4 DOI:10.1109/ICCE.2019.8661979.
-
S. N. Panda, S. Verma, M. Sharma, U. Desai and A. Panda, Smart and Portable IoT Drug Dispensing System for Elderly and Disabled Person, in: 2022 IEEE 7th International Conference on Recent Advances and Innovations in Engineering (ICRAIE), IEEE, 2022: 144–147 DOI:10.1109/ICRAIE56454.2022.10054251.
- B. Tortelli, H. Fujiwara, J. H. Bagel, J. Zhang, R. Sidhu, X. Jiang, N. M. Yanjanin, R. K. Shankar, N. Carillo-Carasco, J. Heiss, E. Ottinger, F. D. Porter, J. E. Schaffer, C. H. Vite and D. S. Ory, Cholesterol homeostatic responses provide biomarkers for monitoring treatment for the neurodegenerative disease Niemann–Pick C1 (NPC1, Hum. Mol. Genet., 2014, 23, 6022–6033, DOI:10.1093/hmg/ddu331.
|
This journal is © The Royal Society of Chemistry 2024 |
Click here to see how this site uses Cookies. View our privacy policy here.