Oxidative potential of atmospheric particulate matter collected in low-income urban settlements in South Africa
Received
5th August 2024
, Accepted 4th November 2024
First published on 7th November 2024
Abstract
Health-related impacts e.g. respiratory and cardiovascular morbidity and mortality, associated with exposure to atmospheric particulate matter (PM) are globally considered important and are not completely understood. Oxidative potential (OP), defined as a measure of the capacity of PM to oxidise target molecules, has been previously proposed as an alternative relevant biological metric in health studies to better quantify toxicological responses associated with PM exposure than aerosol mass alone. Several methods are currently used to assess the oxidative capacity of PM. In this study, the dithiothreitol (DTT) assay was used, which is the most commonly used technique to estimate OP. This assessment is easy-to-operate, low-cost, effective and reproducible. The first step was to modify the DTT methodology based on previous applications, which entailed choosing an appropriate extraction procedure and -setup. The redox activity of size-resolved PM samples collected in three low-income urban settlements in South Africa, i.e. Jouberton, KwaZamokuhle and Zamdela was evaluated and related to their chemical composition through correlation analysis. Furthermore, it was attempted to determine seasonal variations of DTT redox activity through normalisation according to PM mass (DTTm) and sampled volume (DTTv) for outdoor and indoor environments. The results indicated higher redox activity for the finest (<1 μm) particles compared to the coarser particulates (1–10 μm) for both outdoor and indoor environments. DTT redox activity of PM, especially, in the PM1–10 particle size fraction, had strong correlations with elemental (EC) and organic carbon (OC), as well as trace elements and water-soluble inorganic species for outdoor and indoor samples. Possible atmospheric aerosol emission sources suggested from these correlations include primary emissions from domestic- and open biomass burning, vehicles and industrial activities, as well as secondary particle formation (e.g. sulphate).
Environmental significance
Health-related impacts associated with exposure to atmospheric particulate matter (PM) are globally considered important and not completely understood. Oxidative potential (OP), which is a measure of the capacity of PM to oxidise target molecules, has been previously proposed as an alternative relevant biological metric in health studies to better quantify toxicological responses associated with PM exposure. The redox activity of size-resolved indoor and outdoor PM samples collected in three low-income urban settlements in South Africa was evaluated. Higher redox activity was determined for the finest (<1 μm) particles compared to the coarser particulates (1–10 μm) for both outdoor and indoor environments. DTT redox activity of PM, especially, in the PM1-10 particle size fraction, had strong correlations with elemental (EC) and organic carbon (OC), as well as trace elements and water-soluble inorganic species for outdoor and indoor samples. These findings have dramatic implications for the management of air pollution in South Africa, while the modification of the method used to determine OP will simplify the use of this method in other health-related studies.
|
1. Introduction
Recent studies have shown that humans living in cities, especially in developing countries, are most exposed to aerosol or particulate matter (PM) pollution, due to increased industrial activity, energy demand and vehicular emissions.1–3 The health impacts associated with the emissions of these species are of global interest and concern due to increases in emissions of these species in the atmosphere.4–8 Atmospheric pollution related to these species is rapidly becoming one of the leading causes of increased morbidity and mortality in developing countries such as those in Africa, which includes South Africa.4,9 PM can penetrate the respiratory system, which can result in a wide range of impacts on human health that include respiratory- and cardiovascular diseases, premature mortality,10–13 dysfunctions of reproductive and central nervous system (or adverse neurodevelopmental impacts), and cancer.1,10–12 Several epidemiological studies, such as Kampa and Castanas, 2008, and Du et al., 2016, have shown a clear correlation between increased respiratory and cardiovascular morbidity and mortality, and elevated atmospheric PM concentrations.3,6
Health effects resulting from exposure to atmospheric PM are still not completely understood. An important mechanism from which the impacts of atmospheric aerosols on health could be quantified relates to oxidative stress associated with reactive oxygen species (ROS) or free radicals.14–17 Oxidative stress is a biochemical condition where elevated levels of ROS exceed the natural (cellular counteracting) antioxidant capacity, which can cause damages to biological (structural and functional) molecules such as human tissues and cells, lipids, proteins, DNA, carbohydrates and RNA.8–19 ROS may enter the human body in many ways, but it is primarily introduced through inhalation. Aerosol oxidative potential (OP), which is defined as a measure of the capacity of PM to oxidise target molecules, has been previously proposed as an alternative a-cellular relevant biological metric in health studies to better quantify toxicological responses associated with PM exposure than aerosol mass alone.20
Epidemiological studies in which oxidative stress and inflammation are related to cardiovascular morbidity and mortality are limited. Therefore, the OP of particles can be an important biological metric,21,22 since it is related to the chemical composition and size of particulates. Previous studies showed the impacts of aerosols on human health can be related to concentrations of PM10 (particulates with an aerodynamic parameter ≤ 10 μm).23 However, these studies are not ideal from a toxicological and epidemiological perspective, since PM10 also comprise chemical species with low toxicity, e.g. sea salt and crustal species.24–27 More significant impacts on health are associated with fine (PM2.5 – particulates with an aerodynamic parameter ≤ 2.5 μm) and ultrafine (PM1 – particulates with an aerodynamic parameter ≤ 1 μm) aerosols, since these species can diffuse through membranes into human tissue and cells. In addition, these smaller particulates have a larger surface area for exposure to chemical species, which is important in pro-inflammation as indicated through studies on animals and in in vitro tests.9,21 Atmospheric aerosols comprise inorganic and organic compounds, of which a number have studies have indicated the impacts of these chemical species on toxicological responses. Val et al.,9 for instance, conducted an in vitro study on the biological reactivity of urban African particulate matter on human bronchial epithelial cells, as well as their relationship with physical and chemical properties of aerosol species. It was found from the correlation of the gene expression signature with the main chemical compounds in PM that PM-induced responses are mostly associated with organic species (carbonaceous aerosols) and the finest size fraction had the highest toxicity. In addition, the OP is also related to the concentration of PM in the atmosphere and could therefore be considered as a complementary measurement. Although the chemical composition is important, previous studies have showed concentrations to be the primary driver for total OP.
There are several methods used to assess the oxidative capacity of PM, which include cellular assays (e.g. antioxidants depletion assays, antioxidants inactivation assays, and Fenton and Fenton-type reactions), as well as a-cellular assays (e.g. dithiothreitol, DTT, electron spin resonance, ESR, ascorbate acid depletion, AA and reductive acridinium triggering, CRAT).17,20,28 Each of these assays has a different sensitivity to compounds generating ROS. In this study, an a-cellular assay, i.e. DTT, was applied, which is a simple method that uses DTT as a chemical surrogate of cellular antioxidants that do not involve human cells. The consumption of DTT is typically based on the simulation of electron transfer catalysed by PM to form oxygen and generate superoxide.22,29
Previous OP studies utilising DTT used various mediums to extract PM from filters, which include water,20,30–32 dichloromethane33 and methanol.20,28,30,34 However, the recovery rate when using these methods is very low. For example,20,35 and Rattanavaraha et al.30 reported that trace metals, which are important to PM toxicity, might potentially be lost or contaminated during these extraction processes. Therefore, the method to determine DTT activity was modified in this study to be more efficient and simpler. The novelty in this study was to directly incubate filters and 1,4-naphthoquinone (1,4-NQ – used as a positive control with DTT) without any extractant, which, according to the knowledge of the authors, has not been performed before. Note that uncertainty may also exist with our newly developed method due to offline OP measurements after the collection of samples. Indeed, there are a few recent studies that investigated how atmospheric ageing of aerosols impacts the OP of PM using the DTT assay methodology.36–40 There would be a loss of short-lived redox active species in the samples stored for days, weeks or months before they are analysed for OP: the samples might be biochemically less active than those collected and analysed immediately. This would result in an undervaluation of the ROS concentration of the ambient particle bound.41,42 For example, Carlino et al.41 investigated the stability of ROS over samples stored for up to four months and found that the stability of ROS was decreasing with increased storage time. Approximately 60% of ROS in that study were highly reactive and this indicated how quick real-time online ROS analyses need to be considered in the future.
In this study, our newly developed method was applied to different types of filters, namely Teflon™, Quartz and Nucleopore filters, which could also influence the OP measurements. These filter types were used in this study, since it allows measurement of OP of particulates and chemical composition (organic and inorganic species). Finally, this newly DTT method developed at Laboratoire d’Aérologie (Toulouse, France) was applied to outdoor and indoor aerosol samples collected on Nucleopore filters in low-income urban settlements in South Africa.
2. Materials and methods
2.1. Sampling sites and methods
Detailed descriptions of the sampling sites and methods are presented in Segakweng et al.43 PM samples collected at three of the four low-income urban settlements located in the north-eastern interior of South Africa described in Segakweng et al.43 were subjected to OP (DTT) analysis, i.e. ambient aerosols collected at Zamdela (S −26.837, E 27.843) and KwaZamokuhle (S −26.138, E 29.739), and indoor PM samples collected at Jouberton (S −26.906, E 26.584). This is the largest industrialised area in South Africa, holding several pyrometallurgical industries, mines, coal dumps, two large petrochemical plants and a cluster of coal-fired power stations.44 Furthermore, this region is influenced by seasonal regional open biomass burning during the dry season,45 while household combustion is also an important source of atmospheric pollutants in this densely populated region.44,46 The South African Highveld is characterised by a distinct dry and wet season with the dry season usually occurring from mid-May to mid-October, which coincides with the South African winter from June to August. Higher concentrations of atmospheric pollutants during winter are associated with more pronounced inversion layers trapping pollutants near the surface. Ambient measurements performed at KwaZamokuhle and Zamdela were conducted within the framework of the air quality offset programme in South Africa,47 while sampling at Jouberton was conducted as part of the Prospective Household cohort study of Influenza, Respiratory Syncytial virus and other respiratory pathogens community burden and Transmission dynamics in South Africa (PHIRST).48
Winter and summer sampling campaigns were conducted at Zamdela (15 to 30 July 2016 and 09 to 23 March 2017, respectively) and Jouberton (01 August to 16 August 2016 and 18 April to 19 May 2016, respectively), while only a summer campaign was conducted at KwaZamokuhle (23 February until 07 March 2016) due to logistical limitations. Indoor PM samples collected during winter and summer sampling campaigns at Jouberton were used for DTT analysis. However, due to the required sample load for DTT analysis, only ambient aerosol samples collected during winter at Zamdela and only one ambient PM sample collected during summer at KwaZamokuhle could be utilised for DTT analysis.
The ambient and indoor aerosol samples from low-income urban settlements in South Africa were collected on Nucleopore, Teflon and Quartz filters using a set of three cascade Sioutas impactors with 5-stages connected to pumps running in parallel at a flow rate of 9 L min−1. These impactors collected PM in the 2.5–10 μm, 1.0–2.5 μm, 0.50–1.0 μm, 0.25–0.50 μm and <0.25 μm aerodynamic diameter size ranges. One impactor was equipped with Nucleopore filters for the health tests for the DTT redox activity assay. 72 hours ambient aerosol samples were collected at KwaZamokuhle and Zamdela during two-week periods, while at Jouberton, 24 hours indoor PM samples were collected for a duration of seven days in three houses (sampling was only conducted at two of the three houses during the winter campaign due to logistical restraints). The samples were stored in the fridge for two to three years prior to DTT analysis. As previously mentioned, storing sampled filters for long periods can result in the loss of redox active species, which is acknowledged and should be considered when interpreting results presented in this study. However, in view of logistical restraints e.g. effort associated with sampling in these low-income settlements, samples could not be analysed earlier. Results presented can be considered indicative of OP of aerosols collected in these low-income settlements.
2.2. DTT modified method
2.2.1. Introduction of the method.
As previously mentioned, the DTT assay is a chemically redox-active method, which is based on the ability of a PM sample to catalyse electron transfers between DTT and oxygen.30 This assay is a measure of the oxidation of DTT to its disulphide bonded 6-membered ring with the remaining non-oxidised DTT then being reacted with 5,5′-dithio-bis-(2-nitrobenzoic acid) (DTNB). The latter process generate a mixture of the disulphide ring compound and 5-mercapto-2-nitrobenzoic acid (TNB), which is determined through ultraviolet absorption at 412 nm.22 The consumption of DTT by the PM sample is monitored under a set of conditions, which allows the rate to be proportional to the concentration of the catalytically redox-active species contained in the PM sample.22,31 In this study, the DTT method was modified from the more general procedures previously followed e.g. Kumagai et al.29 and Cho et al.22 As mentioned above, in previous studies PM were first extracted with water, dichloromethane or methanol.14,20,30,49,50 In the novel method presented in this study PM particles directly reacted with DTT without any extraction medium (detailed description in subsequent Section 2.2.4). A filter of known mass or a positive control (PC) with 1,4-NQ was immediately incubated with 3 mL of 60 μM DTT solution at 37 °C. The solution was then placed in an ultrasonic bath for 20 minutes, and the intrinsic absorbance (Absi) of the solution was determined with the Infinite F200 PRO TECAN. Similar to the original method, 10 mM DTNB solution was then added to the solution prior to analysis with the Infinite F200 PRO TECAN, which measured the final absorbance of all the elements (Abs), i.e. the absorbance of the 2-nitro-5-thiobenzoic acid (TNB) that is formed during the reaction, at 412 nm. Linear regression of DTT consumption against time can be established and the calculation of absorbance can be expressed as Abs′ = Abs − Absi. The ideal time of the entire experiment/analysis should not exceed 30 min.14
2.2.2. Preparation of reactants for DTT analysis.
All reagents used for the modified DTT assay conducted in this study are presented in Table 1. A 0.1 M phosphate buffer was prepared from K2HPO4, KH2PO4, EDTA and ultrapure water (18 MΩ). A phosphate buffer was used in this study because Pietrogrande et al.28 reported the solution as the solvent of choice with the highest extraction efficiency compared to methanol and Gamble's solution. The solution was dissolved in an ultrasonic bath and the pH of the solution was determined to be 7.3 ± 0.1. This buffer was then used for the preparation of a 5 mM DTT stock solution and a 10 mM DTNB solution. All prepared solutions were stored in a fridge. A 60 μM DTT solution was prepared from the DTT stock solution every day prior to analysis and kept in the fridge in between analyses. A PC solution or instrument control was prepared from 1,4-NQ and ultrapure water (18 MΩ), which was used as the external standard. Details on the concentrations of reagents are described in more detail in (Section 2.2.4).
Table 1 Reagents used for modified DTT assay
Reagent |
Symbol |
Supplier |
Purity |
Potassium phosphate dibasic |
K2HPO4 |
Sigma-Aldrich |
≥98% |
Dithiothreitol (DTT) |
C4H10O2S2 |
Amresco |
|
5,5′-Dithio-bis-(2-nitrobenzoic acid) (DTNB) |
C14H8N2O8S2 |
PanReac AppliChem |
≥98% |
1,4-Naphthoquinone (1,4-NQ) |
C10H6O2 |
Sigma-Aldrich |
97% |
Potassium dihydrogen phosphate |
KH2PO4 |
Alfa Aesar |
99.0% |
Ethylenediaminetetraacetic acid (EDTA) |
C10H16N2O8 |
Alfa Aesar |
99.4+% |
2.2.3. Data calculation and analysis.
In order to assess and compare the OP determined with modified DDT assay in this study to previous studies, the residual DTT (nmol), the mass- and volume-normalised DTT activity (nmol min−1 μg−1 and nmol min−1 m−3), as well as the normalised index of oxidant generation and toxicity (NIOG) were calculated. Residual DTT (nmol) was an indicator of the remaining DTT after the catalytic redox reaction with a decrease in residual DTT corresponding to increased DTT consumption.
where Abs0 is the absorbance associated with DTT added in a blank sample and Abs′ is the absorbance of the formed TNB as mentioned previously.
DTT activity is expressed as the rate of DTT consumption per mass of sample (nmol min−1 μg−1) or per volume of sampled air (nmol min−1 m−3).30 The DTT consumption rate normalised by PM mass (DTTm) represents intrinsic DTT activity of the aerosol OP, while the DTT consumption rate normalised the by total volume of air passing through the filter (DTTv) represents the relevant atmospheric OP.51
where
t is the reaction time (min),
m is the PM mass (μg) and
v is the volume of sampled air.
The NIOG is used to standardise the data obtained from the DTT assay. NIOG is expressed as the ratio of the index of oxidation generation (IOG) of the sample and the IOG of 1,4-NQ (IOG1,4-NQ) that is used as an external standard:
where
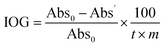
,
t = reaction time (min),
m = sample mass (μg), Abs
0 = absorption when no DTT was reacted, Abs′ = absorption from the remaining DTT after the catalytic redox reaction.
2.2.4. Optimisation of modified DTT method.
Total particulate matter (TPM) samples were collected from a diesel car exhaust on 47 mm Teflon™ and Quartz filters for 24 hours, which were used for validating and/or quantifying the modified DTT methodology. Quartz filters were pre-treated in an oven at 450 °C for 48 hours to remove any impurities. All filters were weighed before and after sampling with the mass of samples collected on Teflon filters ranging between 409 to 904 μg, while the mass of PM collected on Quartz filters ranged from 175 to 758 μg.
The modified DTT assay conducted in this study was optimised by investigating the influence of the following parameters on the calculated OP:
• Concentration and volume of DTT and DTNB;
• Influence of sonication and filtration;
• Use of positive control (1,4-NQ); and
• Influence of reaction time.
2.2.4.1 Concentration and volume of DTT and DTNB.
A sufficient DTT concentration and volume is required to react with the total amount of PM, while the concentration and volume of the DTNB solution should be adequate to consume the residual DTT. An absorbance value of 0.45 is considered a stable absorbance in the pH range between 5.5 and 8.0.31 Therefore, different concentrations of DTT and different volumes of DTNB were evaluated of which the results are listed in Table 2. Similar to previous studies, a fixed DTT volume of 3 mL was chosen, which allows complete immersion of a quarter of the filter for extraction, while a fixed concentration of 10 mM DTNB was used.22 The concentration of DTT was varied from 20–200 μM, while the volume of DTNB ranged from 10–100 μL. It is evident from Table 2 that the absorbance increased with increasing concentration of DTT and for larger volumes of DTNB. According to these tests, the DTT concentration and volume were then fixed at 60 μM and 3 mL, respectively, while a volume and concentration of 10 mM and 50 μL, respectively was used for DTNB (i.e. absorbance of 0.45).31 During these tests, it was also observed that, due to oxidation, the 60 μM DTT solution (prepared from the 5 mM DTT stock solution) is not stable for more than one day. In Fig. 1 the absorbance of a 60 μM DTT solution was monitored for eight days, indicating a daily decline in the measured absorbance. Therefore, in this modified methodology, 5 mM DTT stock solution was prepared once a week, while 60 μM DTT diluted solutions were prepared daily.
Table 2 Absorbance of the reaction products of DTT (3 mL) and DTNB (10 mM)
DTT |
DTNB |
Abs |
20 μM |
10 μL |
0.12989 |
50 μM |
50 μL |
0.24640 |
50 μM |
60 μL |
0.24689 |
60 μM |
50 μL |
0.45284 |
100 μM |
50 μL |
0.51361 |
200 μM |
100 μL |
1.12030 |
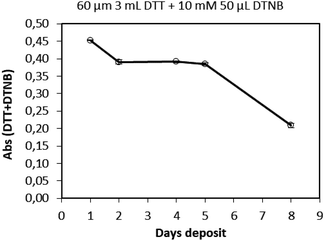 |
| Fig. 1 Absorbance (Abs) of the reaction product of DTT and DTNB over a period of eight days. | |
2.2.4.2 Influence of sonication and filtration.
Three sampled Teflon™ filters (test samples collected from the diesel car exhaust as described above) were cut into four equal parts and separated into two groups to determine the influence of sonication on the reaction with DTT. One-quarter of the sampled filter was immersed in the 3 mL DTT buffer solution, and immediately incubated at 37 °C and shaken for 30 minutes (without sonication). Another quarter of the filter was immersed in the same 3 mL DTT buffer solution in a bottle, and then placed in an ultrasonic bath for 30 minutes, after which it was incubated at 37 °C. The DTNB solution was then added to both reaction solutions and absorbance was measured at 412 nm (each sample was analysed four times). In Fig. 2(a) the residual DTT and (b) DTT activity per mass unit (DTTm) determined for each of the three Teflon™ filters that were sonicated and without sonication are presented. It is evident from Fig. 2 that the DTT response improved when sonicated with significantly more DTT (∼34%) being consumed after sonication compared to ∼4% DTT consumption without sonication. Therefore, all samples were sonicated in the modified DDT assay presented in this study.
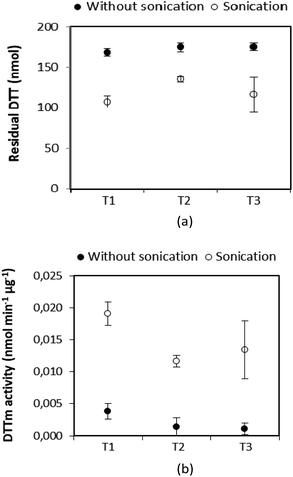 |
| Fig. 2 Comparison of (a) residual DTT and (b) DTTm activity of three Teflon filters (T1, T2 and T3) with or without sonication. The error bars indicate the standard deviations. | |
The residual DTT (a) and DTTm activity (b) determined for sonicated test sampled (diesel car exhaust) Teflon™ filters with and without filtration of the solution are presented in Fig. 3. Equal amounts of the incubated mixtures were filtered through a 0.2 μm syringe filter in order to remove insoluble components prior to adding DTNB. A comparison of filtered and equal amounts of unfiltered samples in Fig. 3 indicates marginally higher residual DTT and lower DTTm activity for filtered samples. Therefore, samples were also filtered in this modified DDT assay conducted in this study to remove insoluble components from the solution. Larger differences between residual DTT and DTTm activity for filtered and unfiltered samples are usually expected according to theory, since any insoluble fraction in the sample could result in light scattering in UV-Vis spectrophotometry. However, it seems that samples extracted in this study either had very low levels of insoluble species or insoluble species did not influence the analytical method. Also indicated in Fig. 3 is the difference between the addition of DTNB after 30- and 60 min reaction time of PM with DTT. Lower DTTm activity was determined for the reaction mixture subjected to DTNB after 60 min. Therefore, the optimum reaction time of 30 min prior to the addition of DTNB was chosen in the modified DTT assay.
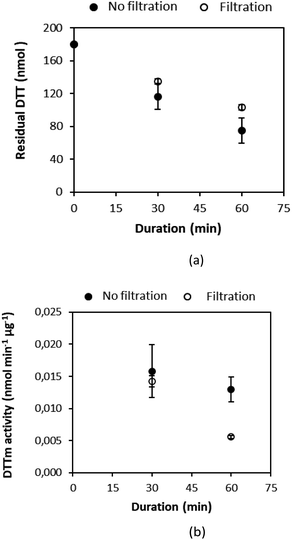 |
| Fig. 3 Comparison of (a) residual DTT and (b) DTTm activity against time (0, 30 and 60 min) on diesel exhaust (Teflon filters) with or without filtration. The error bars indicate the standard deviation. | |
2.2.4.3 Use of positive control (1,4-NQ).
1,4-NQ present in diesel car exhaust particles33,52 has a relatively high content of quinones33 and are commonly used as a reference quinone,31,53–54 while it exhibits high OP and cytotoxicity. The reaction of 1,4-NQ with DTT was also explored to ascertain the efficiency of the DTTm and DTNB concentrations, as well as to normalise the OP and toxicity of samples. In Fig. 4, the residual DTT and DTTm activity for the reaction of DTT with 1,4-NQ is plotted against time. It is evident that the DTTm activity decreased significantly with increased reaction time, which can be attributed to the catalytic redox reaction rates being higher with an increased reaction time. Therefore, these experiments confirmed the 30 minutes reaction time as determined in the previous section.
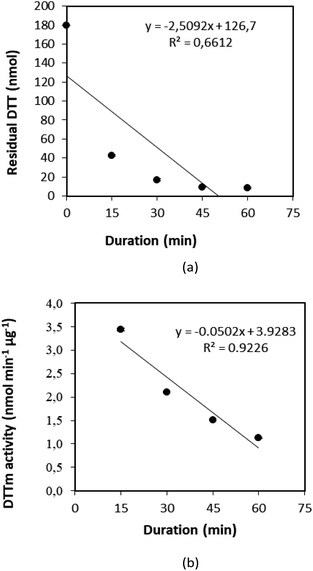 |
| Fig. 4 (a) Residual DTT and (b) DTTm activity against time for the reaction of DTT with the positive control (1,4-NQ). | |
2.2.4.4 Influence reaction time.
Residual DTT and DTTm activity were determined on Quartz and Teflon™ filters at the optimised conditions determined in the previous tests at different reaction times. In addition, the NIOG for each of these experiments was also determined. These results are presented in Fig. 5. It is evident that higher DTTm activity was determined for Quartz filters (Fig. 5b and c) for all the reaction times, which can be attributed to Quartz filters absorbing more DTT during sonication to react with PM. It is also evident that the highest DTTm activity was determined for a reaction time of 30 min, which supports the optimised reaction time determined in the previous tests. DTTm activities determined were in the same order than DTTm activities determined in previous studies using extractants (e.g. Rattanavaraha et al.30 and Geller et al.32).
 |
| Fig. 5 (a) Residual DTT, (b) DTTm activity and (c) NIOG*1000 against time. The error bars show the standard deviation. | |
2.2.5. Filter type.
In addition to the above comparison of TPM collected from a diesel car exhaust on Quartz and Teflon filters, the OP determined with Nucleopore filters were also compared to the OP measured with Quartz filters for indoor and ambient PM2.5 collected at Jouberton (25 samples) and Zamdela (4 samples), respectively, which are presented in Fig. 6. According to the knowledge of the authors, this comparison between OP determined with Quartz and Nucleopore filters has not been done before. With the exception of indoor particulates collected during summer, higher DTTm activity was determined for PM collected on Quartz filters. This suggests that organic species (which are reactive components) were readily extracted from the quartz filter samples during incubation with DTT. Lower DTTm activity values were associated with indoor samples collected on Quartz filters during summer at Jouberton (Fig. 6(a) and sample 4 to 13 in (b)) can possibly be ascribed to the removal of insoluble species during the filtration process. Despite the observed differences between the DTTm activity determined with Quartz and Nucleopore filters, a moderate to relatively strong correlation is determined between DTTm activity of PM collected on these two filter types, i.e. 0.767 and 0.690 for indoor and ambient samples, respectively. This implies that although the type of filter on which PM is collected for the DTT assay has an influence on the absolute DTT value, the relative contribution of PM collected on different filters will be meaningful. As mentioned previously, in this study PM was collected on nucleopore filters specifically for health tests, which were subsequently subjected to DTT redox activity assay.
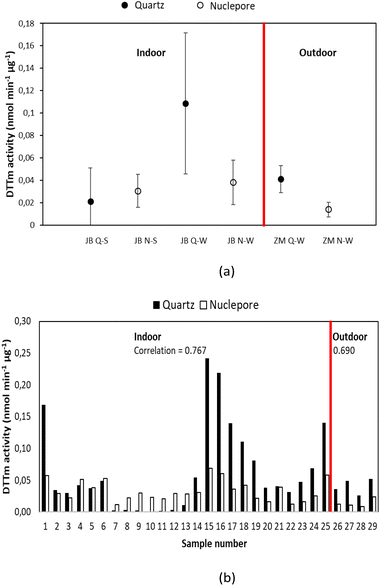 |
| Fig. 6 Comparison of DTTm activity determined for PM2.5 collected on quartz and nucleopore filters at Jouberton (indoor) and Zamdela (ambient) for (a) the entire sampling campaign and (b) individual samples. JB-S = Jouberton summer, JB-W = Jouberton winter and ZM-W = Zamdela winter. | |
Furthermore, DTT redox activity was normalised by total volume (DTTv) and assessed for PM2.5 collected on Quartz and Nucleopore filters (Fig. 7). A significantly higher correlations are observed for DTTv, i.e. correlations of 0.97 and 0.94 for ambient and indoor samples, respectively, which implies that any filter type is suitable for DTTv analysis.
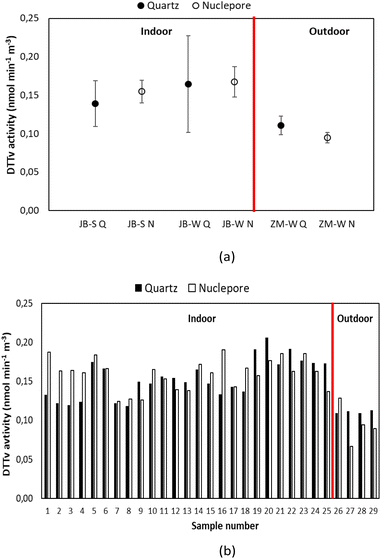 |
| Fig. 7 Impacts of DTT redox activity determined for (a) total volume (mean values) on quartz and nucleopore filter types for PM2.5 collected at all the sites and for (b) individual samples. JB-S = Jouberton summer, JB-W = Jouberton winter and ZM-W = Zamdela winter. | |
2.3. Chemical analysis
Detailed descriptions of the chemical analyses of PM collected at the three low-income urban settlements are presented in Segakweng et al.43 In brief, concentrations of inorganic ions and water-soluble organic acids, organic- and elemental carbon (OC and EC, respectively), and trace elements were determined. Inorganic ionic species measured included sodium (Na+), ammonium (NH4+), potassium (K+), magnesium (Mg2+), and calcium (Ca2+), fluoride (F−), chloride (Cl−), nitrate (NO3−) and sulphate (SO42−), while-soluble organic acids included oxalic-(C2O42−), acetic-(CH3COO−), propionic-(C2H5COO−) and formic acid (COO−). These species were extracted in an ultrasonic bath with ultrapure water (resistivity ≈ 18.2 MΩ) and analysed with a Dionex ICS 3000 ion chromatograph (IC).55,56
Particulate OC and EC were quantified with a G4 ICARUS carbon analyser equipped with a non-dispersive infrared detector-NDIR, following a two-step thermal procedure developed by Cachier et al.57 In this method, one part of the filter is firstly treated with a pre-combustion technique to remove OC to determine EC content and the other part is directly analysed with the carbon analyser to determine total carbon (TC). OC concentration can then be determined as the difference between TC and EC.
Trace elements were extracted from sampled filters with hot acid leaching,58 with the extractant comprising aqueous HNO3 and HCl solutions. The cooled-down extracts were then diluted with deionised water in volumetric flasks and subjected to analysis with an Agilent 7500c inductively coupled plasma mass spectrometer (ICP-MS). A total of 35 trace elements could be detected with ICP-MS analysis, which include Be, B, Na, Mg, Al, P, K, Ca, Ti, V, Cr, Mn, Fe, Cu, Co, Ni, Zn, As, Se, Rb, Sr, Mo, Pd, Ag, Cd, Sb, Ba, Pt, Au, Hg, Tl, Pb, Bi, Th and U. Trace element concentrations below the detection limit of the ICP-MS were considered to have concentrations half the detection limit of the species considered, which is a precautionary assumption that is frequently used in health-related environmental studies (e.g. Van Zyl et al.59).
3. Results and discussions
3.1. DTTm redox activity of PM collected at low-income urban settlements
As indicated above, five PM size fractions were collected in this study (>2.5, 1.0–2.5, 0.50–1.0, 0.25–0.50, and <0.25 μm). However, due to the sample load on the nucleopore filters, it was decided to combine the three smaller size fractions, i.e. PM1 and the two larger size fractions, i.e. PM1–10 for the modified DTT assay. The DTTm activity determined for the ambient and indoor particulates are presented in Fig. 8(a), while the 1
:
1 plot of DTTm activity versus NIOG is presented in Fig. 8(b). The mean DTTm activity values for indoor PM1–10 samples ranged between 0.001 and 0.056 nmol min−1 μg−1, while DTTm activity for indoor PM1 ranged between 0.017 and 0.149 nmol min−1 μg−1. Ambient PM1–10 had DTTm activities that ranged from 0.004–0.116 nmol min−1 μg−1, while DTTm activities of ambient PM1 ranged from 0.019–0.282 nmol min−1 μg−1. In Table 3, the DTTm activities determined in this study are compared with DTTm activities determined in previous studies conducted in other parts of the world. It is evident from Table 3 that the DTTm activities determined in this study for PM collected at low-income urban settlements are in the same range as DTTm activities reported in literature. However, some studies reported DTTm redox activities as high as 0.49 nmol min−1 μg−1 for PM2.5 collected at a peri-urban site in Beijing,60 while DTTm activity values of 0.666, 0.617 and 0.484 nmol min−1 μg−1 were reported for PM0.18, PM2.5 and PM2.5–10 samples collected at an underground train station site in the Netherlands.61
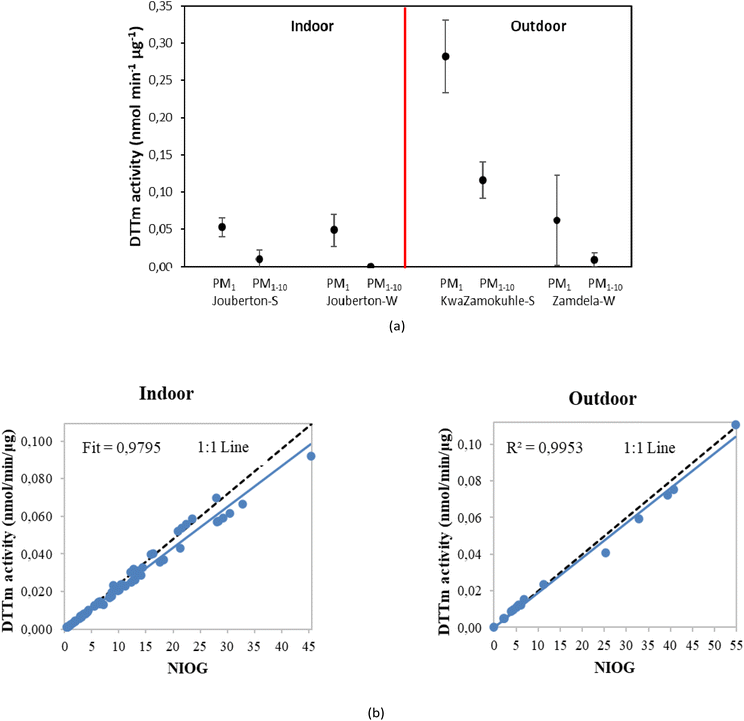 |
| Fig. 8 (a) DTTm activity (nmol min−1 μg−1) for PM nucleopore samples collected from Jouberton (summer and winter), KwaZamokuhle (summer) and Zamdela (winter) for PM1 and PM1–10 size fractions, and (b) correlation between DTTm activity and NIOG for outdoor and indoor samples. | |
Table 3 Contextualisation of DTTm redox activity determined in this studya
Source |
Place |
Particle size |
DTTm activity (nmol min −1 μg−1) |
UT=urban traffic, TSP=total suspended particles.
|
This study |
South Africa (indoor) |
PM1 |
0.017–0.149 (mean = 0.061) |
|
|
PM1–10 |
0.001–0.056 (mean = 0.011) |
|
|
Various |
0.001–0.091 (mean = 0.025) |
|
South Africa (outdoor) |
PM1 |
0.019–0.282 (mean = 0.117) |
|
|
PM1–10 |
0.004–0.116 (mean = 0.036) |
|
|
Various |
0.004–0.150 (mean = 0.038) |
![[thin space (1/6-em)]](https://www.rsc.org/images/entities/char_2009.gif) |
Other countries
|
Pietrogrande et al.28 |
Northern Italy |
PM2.5 |
0.006–0.014 |
Yu et al.62 |
Beijing |
PM2.5 |
0.13 ± 0.1 |
Samara49 |
Thessaloniki. Greece (UT) |
Various |
0.016–0.306 & 0.036–0.271 |
|
Thessaloniki. Greece (UB) |
Various |
0.052–0.459 & 0.012–0.340 |
Chirizzi et al.63 |
Lecce. Southern Italy |
PM2.5 |
0.015 ± 0.008 |
|
|
PM10 |
0.011 ± 0.007 |
Vreeland et al.51 |
Atlanta (side street commute) |
PM2.5 |
0.05 ± 0.03 |
|
Atlanta (highway commute) |
PM2.5 |
0.07 ± 0.07 |
Perrone et al.64 |
Milan. North Italy |
TSP |
0.33–3.43 |
Velali et al.65 |
Thessaloniki. Greece (UB) |
Various |
0.12–0.46 & 0.03–0.34 |
|
Thessaloniki. Greece (UT) |
Various |
0.02–0.31 & 0.05–0.27 |
Shirmohammadi et al.66 |
Los Angeles |
PM2.5 |
0.020–0.045 |
|
|
PM0.18 |
0.020–0.060 |
Samara et al.50 |
Thessaloniki. Greece (UT) |
PM10 |
0.018–0.060 |
Janssen et al.67 |
Netherlands |
PM2.5 |
0.03–0.18 |
Liu et al.60 |
Beijing (peri – urban) |
PM2.5 |
0.11–0.49 (mean = 0.19) |
|
Beijing (central site) |
PM2.5 |
0.09–0.32 (mean = 0.24) |
Saffari et al.68 |
Los Angeles. Basin |
PM2.5 |
0.03–0.11 |
Verma et al.69 |
South-eastern United States |
PM2.5 |
0.005–0.1 |
Charrier and Anastasio70 |
|
PM2.5 |
0.02–0.06 |
Steenhof et al.61 |
Netherlands |
PM0.18 |
0.010–0.666 |
|
|
PM2.5 |
0.030–0.617 |
|
|
PM2.5–10 |
0.022–0.484 |
It is evident from Fig. 8(a) that PM1 had the highest DTTm activity at all three sampling sites, which also corresponds with literature (e.g. Janssen et al.67 and Steenhof et al.61). In addition, the DTTm activities determined for outdoor samples were higher than the DTT values established for indoor PM, except for DTT measured in the PM1–10 size fraction at Zamdela. The highest DTTm activity were determined for ambient PM at KwaZamokuhle in both size fractions. Most studies have shown that ultrafine particles (UPFs) (particles of aerodynamic diameter < ∼0.25 μm) had higher DTTm activity.61,66,71,72 UPFs are strongly associated with impacts of cardiovascular diseases, oxidative stress and systemic inflammation, as well as activation of platelets.73 Josipovic et al.74 (Vaal Triangle, South African study) reported a higher percentage of DTT depletion in UPFs during the wet season and for larger particles during the dry season. Seasonal patterns could not be discerned due to the limitations in the collection of samples (no winter campaign was conducted for KwaZamokuhle), while the sample load of PM collected at Zamdela during summer was too low for DTT assay. No significant differences were observed in DTTm activity determined for indoor samples collected during winter and summer at Jouberton. A strong correlation is observed between DTTm redox activity and NIOG for indoor and ambient PM (R2 = 0.9795 and 0.9953, respectively), which confirms the quality of the DTT assay conducted in this study.
Differences between the OP of outdoor and indoor aerosols have been reported in previous studies. Yang et al.75 indicated the OP of outdoor PM2.5 being twice as high as indoor PM2.5 at Nanjing, China. This was attributed to a decrease in the oxidative reduction of PM during outdoor-to-indoor transport. However, Khurshid et al.76 reported indoor OP (using electron paramagnetic resonance spectrometry assay) being ∼3.5 times higher than that of outdoor OP of aerosols, which were influenced by several factors, such as ventilation rate, temperature, and relative humidity.
3.2. DTTm activity and aerosol chemistry
In Segakweng et al.43 a detailed assessment of the chemical composition of PM1, PM1–2.5 and PM2.5–10 collected in these low-income urban settlements is presented. However, in order to relate the chemical characteristics of PM to the DTTm activities determined in this study, the average concentrations measured for inorganic ions, water-soluble organic compounds, OC, EC and trace elements in PM1 and PM1–10 collected at KwaZamokuhle, Zamdela and Jouberton are presented in Fig. 9 (inorganic ions, water-soluble organic acids, OC and EC) and in Fig. 10 (trace elements). As mentioned above, no PM was collected on Teflon™ filters during the winter campaign at Jouberton due to issues with sampling instruments. As indicated and discussed in detail by Segakweng et al.,43 the highest concentrations of most species (e.g. SO42−, NH4+, water-soluble OAs, EC and OC) were in the PM1 size fraction, while higher levels of these species were generally determined for indoor particulates with the exception of certain important species e.g. SO42−, NO3−, NH4+ and Ca. The highest DTTm activities presented above correspond to the highest concentrations of chemical species being in the PM1 size fraction. Other deductions from the comparison of aerosol chemistry to DTTm activity are not that obvious, which can be attributed to the logistical constraints in this study and complex atmospheric chemistry. In addition, seasonal variability could also no be distinctly established for DTTm activity and for aerosol chemistry.
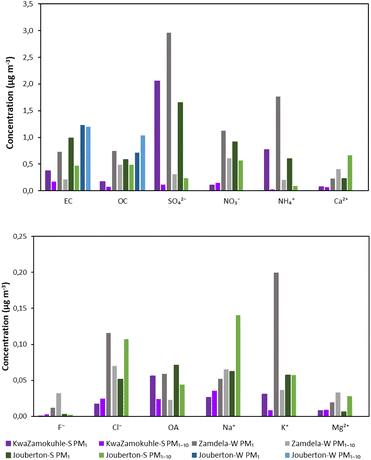 |
| Fig. 9 Mean concentration of EC, OC, inorganic ions, and water-soluble organic acids (OA) at KwaZamokuhle, Zamdela and Jouberton in PM1 and PM1–10 size fractions. | |
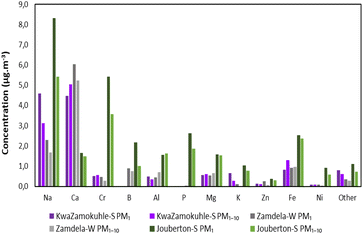 |
| Fig. 10 Mean concentration of trace elements at KwaZamokuhle, Zamdela and Jouberton in PM1 and PM1–10 size fractions. | |
OC/EC, SO42−/EC, EC/TPM (total particulate matter), SO42−/TPM and TE (total trace elements)/TPM ratios are presented in Table 4 for PM1 and PM1–10. Higher SO42−/EC, EC/TPM and SO42−/TPM ratios are observed for PM1 at all sites. In addition, TE/TPM was also higher for the PM1 size fraction apart from of this ratio calculated for KwaZamokuhle. OC/EC ratios were higher in the PM1–10 size fraction except for the OC/EC ratio determined at KwaZamokuhle, which was marginally higher in PM1. As explained in Segakweng et al.,43 higher SO42−/EC and SO42−/TPM ratios observed in outdoor sites indicate the influence of industrial combustion emissions, while higher EC/TPM ratios indicate the impact of domestic and open biomass burning.
Table 4 OC/EC, SO42−/EC, EC/TPM, aSO42−/TPM and TE/TPM ratios in PM1 and PM1–10 size fractions for outdoor (KwaZamokuhle summer and Zamdela winter) and indoor (Jouberton summer and winter) sites
Ratio |
KwaZamokuhle-S |
Zamdela-W |
Jouberton-S |
Jouberton-W |
PM1 |
PM1–10 |
PM1 |
PM1–10 |
PM1 |
PM1–10 |
PM1 |
PM1–10 |
OC/EC |
0.46 |
0.44 |
1.02 |
2.33 |
0.59 |
1.03 |
0.58 |
0.87 |
SO42−/EC |
5.50 |
0.66 |
4.07 |
1.50 |
1.67 |
0.51 |
— |
— |
EC/TPM |
0.05 |
0.03 |
0.03 |
0.01 |
0.03 |
0.01 |
0.04 |
0.02 |
SO42−/TPM |
0.26 |
0.02 |
0.11 |
0.01 |
0.04 |
0.01 |
— |
— |
TE/TPM |
1.67 |
1.85 |
0.45 |
0.39 |
0.79 |
0.48 |
— |
— |
3.3. Statistical correlations between DTTm activity and concentrations of chemical species
DTTm activity for ambient and indoor PM1 and PM1–10 statistically correlates with the chemical species. In these correlations, both the mass normalised DTT activity (nmol min−1 μg−1) and the mass normalised concentrations of chemical species (through calculation of the ratio between the concentrations of chemical species and the total PM concentrations) were used. These correlations are presented in Table 5. Good correlation between DTTm redox activity and chemical species is obtained on the PM1–10 size fraction for both outdoor and indoor measurements. For indoor samples, a good correlation was observed between DTTm activity and EC/PM, TC/PM, F−/PM, NO3−/PM, NH4+/PM and WSI (sum of ionic species)/PM. Indoor (Jouberton summer and winter) also displayed higher OC/EC ratios in the PM1–10 size fraction. Most of the ratios (EC/PM, TC/PM, SO42−/PM, NO3−/PM, K+/PM, OA/PM and overall WSI/PM) for outdoor samples displayed a good correlation with DTTm activity of PM on the PM1–10 size fraction. Note that a strong correlation was obtained between OA/PM for outdoor samples in both size particle fractions as well as EC/PM and TC/PM. This agrees with the OC/EC values observed in both size particle fractions in KwaZamokuhle. Strong correlation between DTTm activity and trace elements was also observed in the PM1–10 size fraction for both outdoor and indoor measurements, with outdoor displaying an important correlation (even redox-active elements such as Cu, Fe and Mn). This is in agreement with the total TE/TPM ratio, which is higher in the PM1–10 for outdoor samples.
Table 5 Correlation between mass normalised DTTm activity, and the ratio between the concentrations of chemical species and the total PM concentrations
To conclude, Table 5 shows that lower correlations are obtained for the PM1 size fraction than for the PM1–10 size fraction, which may be unexpected. However, it is important to mention that fine particles are included in our PM1–10 size fraction with noticeable effects on oxidative potential. Moreover, as it may be seen, better correlations between EC/PM, OC/PM, TC/PM, and OA/PM and DTTm activities for the PM1 size fraction may be observed for outdoor than for indoor samples. This could be due to the different relative contributions of EC and organic aerosol in the samples.
Furthermore, the correlations between DTTm activity and individual chemical species in this study are compared to those of previous studies, which are presented in Table 6 for different size fractions. Cu, Fe and Mn strongly correlate with DTTm activity on the PM1–10 size fraction for outdoor compared to indoor samples, whereas the other redox-active transition elements show negative to moderate positive correlation for both size fractions. Previous studies also reported a good correlation between DTTm activity and redox-active transition elements.77,78 Crustal elements such as Al, K, Mg, Ti and Ca also show good correlation for PM1–10 size particles with outdoor displaying the strongest correlation. The correlation is higher than that reported in previous studies.79 Pietrogrande et al.14 reported moderate to good correlation for crustal elements (Cu, Fe, K, Mn and Ca) ranging from 0.55–0.79 on the PM10 size fraction. A strong correlation between crustal elements and DTTm activity (0.61–0.90, except for Ti) was also reported previously for different extraction methods with no information on particular size fraction.20 Overall, trace elements in this study conducted at low-income informal settlements in South Africa display a good correlation with DTTm activity. Yang et al.79 reported strong correlations (0.92 for total PM with particle size not specified), while Pietrogrande et al.28 indicated good or moderate correlations (0.64) for PM2.5 between the total sum of trace elements and DTTm activity. In contrast, Ntziachristos et al.78 reported weak correlation between metals/elements and DTTm activity (−0.19 to 0.44). Outdoor samples, in this study, displayed the strongest correlation between trace elements and DTTm activity for both size fractions.
Table 6 Comparison between DTT activity correlations with chemical species determined in this study with correlations determined in other studies
|
This study |
(Pietrogrande et al. 2021)28 |
(Yang et al. 2019)79 |
(Pietrogrande et al. 2018)14 |
(Karavalakis et al. 2017)77 |
(Perrone et al. 2016)64 |
(Janssen et al. 2014)20 |
(Yang et al. 2014)14 |
(Ntziachristos et al. 2007)78 |
Indoor |
Outdoor |
P Buffer |
Gamble |
MeOH |
Gasoline direct injection vehicles |
Northern Italy (industrial) |
Background |
Heavy-duty vehicles |
Milan, Norther Italy |
PM2,5 |
PM2,5–10 |
PM10 (PM2,5+2,5–10) |
Met Q |
Met T |
H2O T |
All |
PM0,15 |
PM2,5 |
PM1 |
PM1–10 |
PM1 |
PM1–10 |
PM2,5 |
PM2,5 |
PM2,5 |
|
PM10 |
PM10 |
EC |
0.47 |
0.94 |
0.85 |
0.99 |
0.51 |
0.78 |
0.63 |
— |
— |
— |
— |
0.27 |
0.24 |
0.64 |
0.40 |
0.38 |
0.35 |
0.32 |
0.26 |
0.14 |
−0.18 |
OC |
0.10 |
0.39 |
0.69 |
−0.32 |
0.42 |
0.67 |
0.55 |
— |
— |
— |
— |
−0.30 |
0.69 |
0.45 |
−0.13 |
0.88 |
0.95 |
0.96 |
0.12 |
0.92 |
0.79 |
Al |
0.08 |
0.75 |
−0.27 |
0.99 |
— |
— |
— |
0.18 |
0.25 |
0.35 |
— |
−0.21 |
— |
— |
— |
0.80 |
0.74 |
0.72 |
— |
−0.10 |
−0.67 |
Cr |
0.59 |
0.48 |
−0.61 |
−0.87 |
— |
— |
— |
0.40 |
−0.06 |
−0.13 |
— |
— |
— |
— |
— |
0.57 |
0.41 |
0.46 |
0.65 |
0.53 |
0.86 |
Cu |
0.14 |
0.39 |
−0.45 |
0.99 |
— |
— |
— |
0.20 |
0.58 |
0.69 |
1.00 |
−0.24 |
0.25 |
0.54 |
0.47 |
0.62 |
0.66 |
0.63 |
— |
0.95 |
0.94 |
Fe |
0.39 |
0.59 |
−0.47 |
0.97 |
0.34 |
0.54 |
0.42 |
0.21 |
0.38 |
0.60 |
0.99 |
−0.43 |
0.31 |
0.47 |
0.44 |
0.63 |
0.68 |
0.64 |
— |
0.95 |
0.96 |
K |
−0.13 |
0.86 |
−0.50 |
1.00 |
— |
— |
— |
0.09 |
0.75 |
0.79 |
— |
— |
— |
— |
— |
0.82 |
0.87 |
0.90 |
— |
−0.06 |
−0.69 |
Mn |
0.47 |
0.57 |
−0.67 |
0.99 |
0.36 |
0.61 |
0.47 |
0.24 |
0.61 |
0.68 |
— |
−0.35 |
— |
— |
— |
0.67 |
0.65 |
0.61 |
— |
0.90 |
0.78 |
Ni |
0.24 |
0.40 |
−0.63 |
−0.28 |
— |
— |
— |
0.32 |
0.36 |
0.41 |
— |
0.18 |
0.01 |
0.25 |
0.34 |
0.15 |
0.13 |
0.20 |
— |
0.55 |
−0.46 |
Pb |
0.24 |
−0.07 |
0.86 |
1.00 |
0.27 |
0.87 |
0.76 |
0.08 |
0.3 |
0.68 |
— |
−0.52 |
— |
— |
— |
0.33 |
0.56 |
0.54 |
— |
0.95 |
0.88 |
Ti |
0.30 |
0.74 |
−0.30 |
0.98 |
— |
— |
— |
0.55 |
— |
— |
— |
— |
— |
— |
— |
0.34 |
0.09 |
0.20 |
— |
0.66 |
0.67 |
V |
0.35 |
0.62 |
−0.69 |
−0.97 |
0.43 |
0.47 |
0.68 |
0.29 |
0.09 |
0.23 |
— |
−0.46 |
−0.11 |
0.27 |
0.51 |
−0.05 |
−0.10 |
−0.05 |
— |
0.32 |
0.19 |
Zn |
0.46 |
0.52 |
−0.01 |
0.89 |
0.08 |
0.44 |
0.23 |
0.41 |
0.51 |
0.68 |
— |
−0.40 |
— |
— |
— |
0.63 |
0.68 |
0.67 |
— |
0.93 |
0.52 |
Ca |
−0.19 |
0.58 |
0.86 |
0.99 |
— |
— |
— |
0.18 |
0.50 |
0.55 |
— |
−0.19 |
— |
— |
— |
— |
— |
— |
— |
0.55 |
−0.62 |
P |
0.67 |
−0.18 |
−0.69 |
−0.99 |
— |
— |
— |
0.61 |
— |
— |
— |
— |
— |
— |
— |
— |
— |
— |
— |
— |
— |
Na |
0.75 |
0.58 |
0.00 |
1.00 |
— |
— |
— |
0.04 |
— |
— |
— |
— |
— |
— |
— |
— |
— |
— |
— |
−0.66 |
0.03 |
Mg |
0.37 |
0.40 |
0.89 |
0.97 |
— |
— |
— |
0.46 |
0.56 |
0.61 |
— |
— |
— |
— |
— |
— |
— |
— |
— |
— |
−0.52 |
Mo |
0.51 |
0.68 |
−0.65 |
−0.99 |
— |
— |
— |
0.43 |
— |
— |
— |
−0.55 |
— |
— |
— |
— |
— |
— |
— |
— |
— |
Pd |
−0.14 |
0.70 |
−0.35 |
0.95 |
— |
— |
— |
0.16 |
— |
— |
— |
— |
— |
— |
— |
— |
— |
— |
— |
— |
— |
Sr |
−0.20 |
0.69 |
0.51 |
0.99 |
— |
— |
— |
0.32 |
0.41 |
0.45 |
— |
— |
— |
— |
— |
— |
— |
— |
— |
0.74 |
0.70 |
Co |
0.22 |
0.16 |
−0.59 |
−0.19 |
— |
— |
— |
0.04 |
— |
— |
— |
−0.22 |
— |
— |
— |
— |
— |
— |
— |
— |
— |
As |
0.08 |
0.70 |
−0.70 |
−0.66 |
— |
— |
— |
0.02 |
— |
— |
— |
−0.13 |
— |
— |
— |
— |
— |
— |
— |
— |
— |
Ag |
0.20 |
−0.04 |
−0.18 |
0.29 |
— |
— |
— |
0.26 |
— |
— |
— |
— |
— |
— |
— |
— |
— |
— |
— |
— |
— |
Sb |
0.12 |
0.70 |
−0.49 |
−0.78 |
— |
— |
— |
0.68 |
— |
— |
— |
— |
— |
— |
— |
— |
— |
— |
— |
— |
— |
Rb |
0.17 |
0.89 |
−0.38 |
0.99 |
— |
— |
— |
0.01 |
0.83 |
0.85 |
— |
— |
— |
— |
— |
— |
— |
— |
— |
— |
— |
Cd |
−0.29 |
0.48 |
0.15 |
−0.12 |
— |
— |
— |
— |
— |
— |
— |
−0.46 |
— |
— |
— |
— |
— |
— |
— |
— |
— |
Ba |
−0.15 |
0.72 |
0.92 |
0.98 |
— |
— |
— |
— |
— |
— |
— |
−0.21 |
— |
— |
— |
— |
— |
— |
— |
0.89 |
0.92 |
Total TE |
0.34 |
0.65 |
0.83 |
0.99 |
0.24 |
0.64 |
0.40 |
0.92 |
— |
— |
— |
— |
— |
— |
— |
— |
— |
— |
−0.19 |
0.44 |
−0.12 |
NO3–/NO3 |
−0.07 |
0.80 |
−0.73 |
0.87 |
0.24 |
0.36 |
0.31 |
— |
0.40 |
0.45 |
— |
−0.11 |
0.51 |
0.47 |
0.16 |
— |
— |
— |
−0.45 |
−0.63 |
−0.81 |
SO42−/SO4 |
−0.37 |
0.41 |
−0.67 |
0.88 |
0.45 |
0.38 |
0.50 |
— |
0.47 |
0.7 |
— |
0.16 |
0.41 |
0.59 |
0.46 |
— |
— |
— |
−0.08 |
−0.75 |
−0.80 |
F− |
−0.19 |
0.83 |
−0.78 |
−0.07 |
— |
— |
— |
— |
— |
— |
— |
−0.47 |
— |
— |
— |
— |
— |
— |
— |
— |
— |
Cl− |
0.25 |
0.33 |
−0.95 |
−0.53 |
0.47 |
0.40 |
0.47 |
— |
0.09 |
0.17 |
— |
−0.35 |
— |
— |
— |
— |
— |
— |
— |
— |
— |
Na+ |
0.05 |
0.37 |
−0.33 |
0.20 |
— |
— |
— |
— |
0.32 |
0.24 |
— |
— |
— |
— |
— |
— |
— |
— |
— |
— |
— |
NH4+ |
−0.20 |
0.83 |
−0.93 |
0.42 |
— |
— |
— |
— |
0.46 |
0.67 |
— |
0.06 |
— |
— |
— |
— |
— |
— |
— |
— |
— |
K+ |
0.08 |
0.49 |
−0.94 |
0.70 |
— |
— |
— |
— |
0.77 |
0.84 |
— |
−0.39 |
— |
— |
— |
— |
— |
— |
— |
— |
— |
Mg2+ |
0.09 |
0.41 |
−0.60 |
−0.09 |
— |
— |
— |
— |
0.57 |
0.62 |
— |
— |
— |
— |
— |
— |
— |
— |
— |
— |
— |
Ca2+ |
−0.20 |
0.44 |
−0.49 |
0.22 |
— |
— |
— |
— |
0.52 |
0.57 |
— |
−0.27 |
— |
— |
— |
— |
— |
— |
— |
— |
— |
OA |
0.10 |
0.37 |
0.93 |
0.98 |
— |
— |
— |
— |
— |
— |
— |
— |
— |
— |
— |
— |
— |
— |
— |
— |
— |
WSI |
−0.27 |
0.67 |
−0.85 |
0.73 |
— |
— |
— |
— |
0.57 |
0.73 |
— |
— |
— |
— |
— |
— |
— |
— |
— |
— |
— |
Outdoor and indoor samples show good correlation between EC/PM and DTTm activity compared to OC/PM. This agrees with the study of Janssen et al.67 for PM2.5–10 and PM10, Pietrogrande et al.28 for PM2.5 and Ntziachristos et al.78 for various sizes and is the opposite from findings from other studies.20 Good correlation between EC/PM and DTTm activity is stronger on the PM1–10 size fraction with outdoor displaying a strong correlation in both size fractions. A good correlation between DTTm activity and OC/PM is observed in the PM1 size fraction (0.69) for outdoor measurements, which is interesting as it is similar to that of previous studies of 0.67 for PM2.5 (ref. 28) and 0.69 for PM2.5.20 Puthussery et al.42 found a good correlation between DTTm activity (intrinsic OP, mass normalised DTT activity) and oxygenated organic aerosol species (aged aerosols) (r = 0.79), a family of CHO>1 (r = 0.80), K (r = 0.70) and SO42− (r = 0.68).
Finally, as stated above, good correlation between WSI and DTTm activity is mainly observed in the PM1–10 size fraction. This agrees with literature where SO42−, HN4+, K+, Mg2+, Ca2+ and overall WSI showed moderate to good correlation (0.52–0.84) for PM10,14 while correlations in previous studies were negative to moderate.64,67,78 The good correlation between DTTm activity and OA/PM for both size fractions observed in this study is not reported in previous studies.
3.4. Comparison between DTTv and DTTm
The highest DTTv (representing the atmospheric aerosol OP) activity is also displayed in the PM1 size fraction at all three sites (Fig. 11). For indoor aerosols, DTTv is marginally higher in winter compared to summer in both size fractions. This could be due to increased grass and waste burning practices in Jouberton that occur during either season. As previously mentioned, not much can be said about seasonality in the outdoor environments due to sampling logistics and less sample loading on some of the filters. Comparisons between DTTm (DTT consumption rate normalised by PM mass) and DTTv at three low-income urban settlements indicate that both DTTm and DTTv are higher in the PM1 size fraction for all the sites. In addition, DTTv are higher than DTTm in all samples collected at the three sites in both size fractions. Pietrogrande et al.28 also reported higher DTTv values (ranging from 0.02–0.41 nmol min−1 m3) compared to DTTm (ranging from 0.001–0.021 nmol min−1 μg−1) for ambient PM2.5 samples extracted with a different solvent mixture.
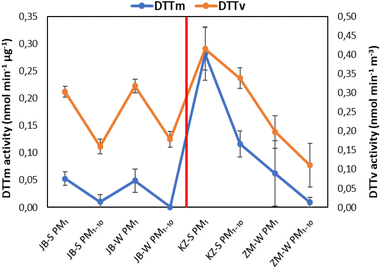 |
| Fig. 11 Comparison of DTTm and DTTv in low-income urban settlements for outdoor and indoor samples. JB-S = Jouberton summer, JB-W = Jouberton winter, KZ-S = KwaZamokuhle summer and ZM-W = Zamdela winter. | |
DTTv for outdoor and indoor PM1 and PM1–10 is statistically correlated with the chemical species. These correlations are presented in Table 7. Good correlations between DTTv and some chemical species are obtained on the PM1 size fraction for both outdoor and indoor measurements. For indoor samples, a good correlation is observed between DTTv and Cl− (0.60) and Mg2+ (0.65) on the PM1 size fraction and SO42− (0.64) and Ca2+ (0.63) on the PM1–10 size fraction. No correlation is observed between DTTv and carbonaceous aerosols for indoors. Most of the ions (SO42−, NO3−, Na+, NH4+, K+, OA and overall WSI) for outdoor samples display good to strong correlation with DTTv on the PM1 size fraction ranging from 0.60 to 1.0. Calas et al.80 reported good correlation between few ionic species (Cl−, NO3− and K+) and DTTv of ambient aerosols in the PM10 size fraction ranging from 0.60 to 0.83. It is noted that strong correlation of 0.79 is obtained between OC and DTTv for outdoor samples on the PM1–10 size particle fraction, whereas the correlation is a bit lower in the PM1 size fraction, as shown for DTTm in Section 3.3. A strong correlation was also previously observed between DTTv and OC (ranging from 0.75–0.86) and EC (0.64–0.93) for PM10 size particles.80 Good correlations between DTTv and trace elements are observed in both size particle fractions (PM1 and PM1–10) for outdoor measurements, while for indoor measurements, good correlation is observed on the PM1 size fraction. Outdoor displaying important correlation for redox-active elements such as Cu (0.79), Fe (0.60) and Mn (0.70). Calas et al.80 also obtained good correlation for redox-active elements such as Cu (0.77), Fe (0.76) and Mn (0.68) in one of the urban French sampling sites (Grenoble).
Table 7 Correlation between volume normalised DTT activity and the concentrations of chemical species
4. Conclusions
The DTT redox activity methodology was successfully modified from previous studies, while the influence of concentration, volume, time, filter type, sonication and filtration were investigated. The optimum DTT concentration and volume determined were 60 μM and 3 mL, respectively, while the DTNB concentration and volume were fixed at 10 mM and 50 μL, respectively. The reaction was performed with sonication and filtration at a reaction time of 30 minutes. Quartz filters displayed higher DTT activity, which therefore indicated that this type of filter is satisfactory for determining DTTm, whereas any filter is adequate to establish DTTv. DTTm activities determined were in the same order than DTTm activities determined in previous studies using extractants.
The DTT activity could be determined for size-segregated PM samples collected at three low-income urban settlements in South Africa, i.e. indoor samples at Jouberton during winter and summer, and outdoor samples collected during summer and winter at KwaZamokuhle and Zamdela, respectively. DTTm activity was higher in the PM1 size fraction for all three sites, which suggested that particles of the coarser mode are less enriched with DTT-active compounds compared to that of the finest (ultrafine) particles. DTTm activity correlated with most of the chemical species on the PM1–10 particle fraction, especially for outdoor samples. DTTm activity was strongly correlated with EC (more than OC), indicating the impact of vehicular emissions and other combustion sources on the OP of PM. There was also a strong correlation between DTTm activity and trace elements in general (except for PM1 indoors), while inorganic ions only revealed strong correlation in the PM1–10 size fraction of outdoor and indoor particulates. Results determined for outdoor particles suggest that the OP of PM is influenced by a combination of different contribution sources, including primary emissions such as traffic or vehicular emissions (Fe, Ca, Pb, Mg, EC), biomass burning (EC, K, Rb), industrial or factory (Cu, Zn, NO3−, SO42−, Ca2+, NH4+, Cl−), as well as secondary particle formation (e.g. SO42−). The oxidative potential of PM is also affected by domestic and biomass burning (e.g. OC, EC and OA) from outdoor atmospheric aerosols. OP of PM in an indoor environment (e.g. in Jouberton) may be impacted by different source emissions such as domestic and biomass burning (carbonaceous aerosols), industrial emissions (e.g. Cu, Zn, NO3−, SO42−, Ca2+, NH4+, Cl−), vehicle emissions and unpaved roads (e.g. Fe, Ca, Mn, Mg, Al, Ti, K).
DTTv showed good correlations with most of the chemical species on the PM1 particle fraction, especially for outdoor samples. DTTv activity was strongly correlated with OC (higher than EC), indicating the impact of combustion emissions. There was also a strong correlation between DTTv and trace elements in general (except for indoors), while inorganic ions revealed strong correlation in the PM1 size fraction of outdoor particulates. In conclusion, variation of DTTm and DTTv values is coherent with that of aerosol chemical composition.
The technique used in this study to relate the potential impact of particulates on human health proved to be effective in establishing the link between atmospheric pollutants and sources and human health. The long-term monitoring of DTT redox activity could be a strong and easy – to – set up indicator to measure the health impact of emission mitigations, especially for decision-makers. However, further research is essential, especially in South Africa, which include assessment of seasonal patterns on DTT redox activity. It would be also interesting to investigate the DTTv on personal exposure samples because it outlines the real exposure of atmospheric aerosols to the population. Other proxy methods to relate the impacts of aerosol on human health could also be considered. Finally, future human health studies, including both in vitro and in vivo measurements involving humans, would also be needed in South Africa, to improve the assessment of the impacts of atmospheric pollution on human health in terms of diseases, mortality, etc., and the link with oxidative potential and inflammatory impacts.
Data availability
As soon as the manuscript is approved for publication and a permanent DOI number is obtained for the dataset, it will be indicated in the manuscript.
Author contributions
CKS, CL and PGvZ were the main investigators in this study and wrote the manuscript. CKS conducted this study as part of her PhD degree, as well as performed most of the experimental work and data processing. The project was led by PGvZ, CL and JPB, which were also study leaders of the PhD. SG, EG, CD and BG assisted with analysis of aerosols samples, while MJ and BL assisted with particulate sample collection. RPB and SJP provided infrastructure for sampling campaigns. Conceptual contributions were made by KJ and TX.
Conflicts of interest
There are no conflicts to declare.
Acknowledgements
The financial assistance of the National Research Foundation (NRF) towards this research is hereby acknowledged. Atmospheric Research in Southern Africa and Indian Ocean (ARSAIO) project is also acknowledged for its contribution to this study. The Prospective Household cohort study of Influenza, Respiratory Syncytial virus and other respiratory pathogens community burden and Transmission dynamics in South Africa (PHIRST) study is also acknowledged. This publication forms part of the output of the Biogeochemistry Research Infrastructure Platform (BIOGRIP) of the Department of Science and Innovation of South Africa. Mr Jacques Adon is also thanked for his assistance with OC and EC analysis, as well as Mr Johan Hendriks for conducting ICP-MS analysis.
References
- E. S. Manisalidis, A. Stavropoulos and E. Bezirtzoglou, Environmental and health impacts of air pollution: A Review, Front. Public Health, 2020, 8, 14 CrossRef PubMed.
- L. T. Molina, T. Zhu, W. Wan and B. R. Gurjar, Impacts of megacities on air quality: challenges and opportunities, Oxford Res. Environ. Sci., 2020 DOI:10.1093/acrefore/9780199389414.013.5.
- M. Kampa and E. Castanas, Human health effects of air pollution, Environ. Pollution, 2008, 151, 362–367 CrossRef CAS PubMed.
- S. Keita, C. Liousse, E.-M. Assamoi, T. Doumbia, E. T. N'Datchoh, S. Gnamien, N. Elguindi, C. Granier and V. Yoboué, African anthropogenic emissions inventory for gases and particles from 1990 to 2015, Earth Syst. Sci. Data, 2021, 13, 3691–3705 CrossRef.
- R. B. Hamanaka and G. M. Mutlu, Particulate matter air pollution: Effects on the cardiovascular system, Front. Endocrinol., 2018, 9, 680 CrossRef.
- Y. Du, X. Xu, M. Chu, Y. Guo and J. Wang, Air particulate matter and cardiovascular disease: The Epidemiological, Biomedical and Clinical Evidence, J. Thorac. Dis., 2016, 8, E8 Search PubMed.
- C. Liousse, E. Assamoi, P. Criqui, C. Granier and R. Rosset, African combustion emission explosive growth from 2005 to 2030, Environ. Res. Lett., 2014, 9, 1–10 Search PubMed.
- J. Löndahl, E. Swietlicki, E. Lindgren and S. Loft, Aerosol exposure versus aerosol cooling of climate: What is the optimal emission reduction strategy for human health?, Atmos. Chem. Phys., 2010, 10, 9441–9449 CrossRef.
- S. Val, C. Liousse, E. H. T. Doumbia, C. Galy-Lacaux, H. Cachier, N. Marchand, A. Badel, E. Gardrat, V. Sylvestre and A. Baeza-Squiban, Physico-chemical characterization of African urban aerosols (Bamako in Mali and Dakar in Senegal) and their toxic effects in human bronchial epithelial cells: Description of a worrying situation, Part. Fibre Toxicol., 2013, 10, 10 CrossRef CAS.
- T. Fang, V. Verma, J. T. Bates, J. Abrams, M. Klein, M. J. Strickland, S. E. Sarnat, H. H. Chang, J. A. Mulholland, P. E. Tolbert, A. G. Russell and R. J. Weber, Oxidative potential of ambient water-soluble PM2.5 in the Southeastern United States: Contrasts in sources and health associations between ascorbic Acid (AA) and dithiothreitol (DTT) assays, Atmos. Chem. Phys., 2016, 16, 3865–3879 CrossRef CAS.
- N. A. H. Janssen, M. Strak, A. Yang, B. Hellack, F. J. Kelly, T. A. J. Kuhlbusch, R. M. Harrison, B. Brunekreef, F. R. Cassee, M. Steenhof and G. Hoek, Associations between three specific a-cellular measures of the oxidative potential of particulate matter and markers of acute airway and nasal inflammation in healthy volunteers, Occup. Environ. Med., 2015, 72, 49–56 CrossRef PubMed.
- X. Zhu, Y. Liu, Y. Chen, C. Yao, Z. Che and J. Cao, Maternal exposure to fine particulate matter (PM2.5) and pregnancy outcomes: A meta-analysis, Environ. Sci. Pollut. Res., 2015, 22, 3383–3396 CrossRef CAS.
- A. S. Ibrahim and F. I. Habbani, Black carbon aerosols impact of Khartoum Petroleum Refinery at Khartoum State, Sudan J. Sci., 2013, 5, 69–83 Search PubMed.
- M. C. Pietrogrande, C. Dalpiaz, R. Dell'Anna, P. Lazzeri, F. Manarini, M. Visentin and G. Tonidandel, Chemical composition and oxidative potential of atmospheric coarse particles at an industrial and urban background site in the Alpine Region of Northern Italy, Atmos. Environ., 2018, 191, 340–350 CrossRef CAS.
- J. T. Bates, R. J. Weber, J. Abrams, V. Verma, T. Fang, M. Klein, M. J. Strickland, S. E. Sarnat, H. H. Chang, J. A. Mulholland, P. E. Tolbert and A. G. Russell, Reactive oxygen species generation linked to sources of atmospheric particulate matter and cardiorespiratory effects, Environ. Sci. Technol., 2015, 49, 13605–13612 CrossRef CAS PubMed.
- R. O. Quintana-Belmares, E. Alfaro-Moreno, C. M. García-Cuéllar, V. Gómez-Vidales, I. Vázquez-López, M. de J. Salmón-Salazar, I. Rosas-Pérez and Á. R. Osornio-Vargas, Evaluation of the oxidative potential of urban PM and its relation to in vitro induced DNA damage: A spatial and temporal comparison, Rev. Int. Contam. Ambiental, 2015, 31, 145–154 CAS.
- V. Verma, T. Fang, L. Xu, R. E. Peltier, A. G. Russell, N. L. Ng and R. J. Weber, Organic aerosols associated with the generation of reactive oxygen species (ROS) by water-soluble PM2.5, Environ. Sci. Technol., 2015, 49, 4646–4656 CrossRef CAS.
- R. J. Delfino, N. Staimer and N. D. Vaziri, Air pollution and circulating biomarkers of oxidative stress, Air Qual., Atmos. Health, 2011, 4, 37–52 CrossRef CAS PubMed.
- R. J. Delfino, C. Sioutas and S. Malik, Potential role of ultrafine particles in associations between airborne particle mass and cardiovascular health, Environ. Health Perspect., 2005, 113, 934–946 CrossRef PubMed.
- A. Yang, A. Jedynska, B. Hellack, I. Kooter, G. Hoek, B. Brunekreef, T. A. J. Kuhlbusch, F. R. Cassee and N. A. H. Janssen, Measurement of the oxidative potential of PM2.5 and its constituents: The effect of extraction solvent and filter type, Atmos. Environ., 2014, 83, 35–42 CrossRef CAS.
- P. J. A. Borm, F. Kelly, N. Künzli, R. P. F. Schins and K. Donaldson, Oxidant generation by particulate matter: From biologically effective dose to a promising, novel metric, Occup. Environ. Med., 2007, 64, 73–74 CrossRef PubMed.
- A. K. Cho, C. Sioutas, A. H. Miguel, Y. Kumagai, D. A. Schmitz, M. Singh, A. Eiguren-Fernandez and J. R. Froines, Redox activity of airborne particulate matter at different sites in the Los Angeles Basin, Environ. Res., 2005, 99, 40–47 CrossRef CAS PubMed.
- C. Pope and D. Dockery, Epidemiology of particle effects, Air Pollut. Health, 1999, 673–705 Search PubMed.
- N. M. Hamdan, H. Alawadhi and M. Shameer, Physicochemical characterization and seasonal variations of PM10 aerosols in a harsh environment, Front. Environ. Sci., 2021, 9, 1–13 Search PubMed.
- E. S. Galvão, M. T. D. Orlando, J. M. Jane Meri Santos and A. T. Lima, Uncommon chemical species in PM2.5 and PM10 and its potential use as industrial and vehicular markers for source apportionment studies, Chemosphere, 2020, 240, 124953 CrossRef.
- K. Donaldson, L. Tran, L. A. Jimenez, R. Duffin, D. E. Newby, N. Mills, W. MacNee and V. Stone, Combustion-derived nanoparticles: A review of their toxicology following inhalation exposure, Part. Fibre Toxicol., 2005, 2, 1–14 CrossRef PubMed.
- F. J. Kelly, Oxidative Stress: Its role in air pollution and adverse health effects, Occup. Environ. Med., 2003, 60, 612–616 CrossRef.
- M. C. Pietrogrande, D. Bacco, A. Trentini and M. Russo, Effect of filter extraction solvents on the measurement of the oxidative potential of airborne PM2.5, Environ. Sci. Pollut. Res., 2021, 28, 29551–29563 CrossRef CAS PubMed.
- Y. Kumagai, S. Koide, K. Taguchi, A. Endo, Y. Nakai, T. Yoshikawa and N. Shimojo, Oxidation of proximal protein sulfhydryls by phenanthraquinone, a component of diesel exhaust particles, Chem. Res. Toxicol., 2002, 15, 483–489 Search PubMed.
- W. Rattanavaraha, E. Rosen, H. Zhang, Q. Li, K. Pantong and R. M. Kamens, The reactive oxidant potential of different types of aged atmospheric particles: An outdoor chamber study, Atmos. Environ., 2011, 45, 3848–3855 CrossRef CAS.
- Q. Li, A. Wyatt and R. M. Kamens, Oxidant generation and toxicity enhancement of aged-diesel exhaust, Atmos. Environ., 2009, 43, 1037–1042 CrossRef CAS.
- M. D. Geller, L. Ntziachristos, A. Mamakos, Z. Samaras, D. A. Schmitz, J. R. Froines and C. Sioutas, Physicochemical and redox characteristics of particulate matter (PM) emitted from gasoline and diesel passenger cars, Atmos. Environ., 2006, 40, 6988–7004 CrossRef CAS.
- A. K. Cho, E. Di Stefano, Y. You, C. E. Rodriguez, D. A. Schmitz, Y. Kumagai, A. H. Miguel, A. Eiguren-Fernandez, T. Kobayashi, E. Avol and J. R. Froines, Determination of four quinones in diesel exhaust particles, SRM 1649a, and atmospheric PM 2.5 Special Issue of Aerosol Science and Technology on Findings from the Fine Particulate Matter Supersites Program, Aerosol Sci. Technol., 2004, 38, 68–81 CrossRef CAS.
- N. A. H. Janssen, A. Yang, M. Strak, M. Steenhof, B. Hellack, M. E. Gerlofs-Nijland, T. Kelly, F. Kuhlbusch, R. Harrison, B. Brunekreef, G. Hoek and F. Cassee, Oxidative potential of particulate matter collected at sites with different source characteristics, Sci. Total Environ., 2014, 472, 572–581 CrossRef CAS.
- Q. Li, J. Shang, J. Liu, W. Xu, X. Feng, R. Li and T. Zhu, Physicochemical characteristics, oxidative capacities and cytotoxicities of sulfate-coated, 1,4-NQ-coated and ozone-aged black carbon particles, Atmos. Res., 2015, 153, 535–542 CrossRef CAS.
- M. C. Pietrogrande, M. Russo and E. Zagatti, Review of PM oxidative potential measured with acellular assays in urban and rural sites across Italy, Atmosphere, 2019, 10, 626 CrossRef CAS.
- D. Paraskevopoulou, A. Bougiatioti, I. Stavroulas, T. Fang, M. Lianou, E. Liakakou, E. Gerasopoulos, R. Weber, A. Nenes and N. Mihalopoulos, Yearlong variability of oxidative potential of particulate matter in an urban Mediterranean environment, Atmos. Environ., 2019, 206, 183–196 CrossRef CAS.
- Y. Wang, M. Wang, S. Li, H. Sun, Z. Mu, L. Zhang, Y. Li and Q. Chen, Study on the oxidation potential of the water-soluble components of ambient PM2.5 over Xi’an, China: Pollution levels, source apportionment and transport pathways, Environ. Int., 2020, 136, 105515 CrossRef CAS PubMed.
- S.-H. Oh, M. Song, J. J. Schauer, Z.-H. Shon and M.-S. Bae, Assessment of long-range oriented source and oxidative potential on the South-west shoreline, Korea: Molecular marker receptor models during shipborne measurements, Environ. Pollut., 2021, 281, 116979 CrossRef CAS.
- L. J. Borlaza, S. Weber, A. Marsal, G. Uzu, V. Jacob, J.-L. Besombes, M. Chatain, S. Conil and J.-L. Jaffrezo, Nine-year trends of PM10 sources and oxidative potential in a rural background site in France, Atmos. Chem. Phys., 2022, 22, 8701–8723 CrossRef CAS.
- A. Carlino, M. P. Romano, M. G. Lionetto, D. Contini and M. R. Guascito, An overview of the automated and on-line systems to assess the oxidative potential of particulate matter, Atmosphere, 2023, 14(256), 1–15 Search PubMed.
- J. V. Puthussery, A. Singh, P. Rai, D. Bhattu, V. Kumar, P. Vats, N. Rastogi, J. G. Slowik, D. Ganguly, A. S. H. Prevot, S. N. Tripathi and V. Verma, Real-time measurements of PM2.5 oxidative potential using a dithiothreitol assay in Delhi, India, Environ. Sci. Technol. Lett., 2020, 7, 504–510 CrossRef CAS.
- C. K. Segakweng, P. G. Van Zyl, C. Liousse, J. P. Beukes, J.-S. Swart, E. Gardrat, M. Dias-Alves, B. Language, R. P. Burger and S. J. Piketh, Measurement report: Size-resolved chemical characterisation of aerosols in low-income urban settlements in South Africa, Atmos. Chem. Phys., 2022, 22, 10291–10317 CrossRef CAS.
- T. L. Laban, P. G. Van Zyl, J. P. Beukes, V. Vakkari, K. Jaars, N. Borduas-Dedekind, M. Josipovic, A. M. Thompson, M. Kulmala and L. Laakso, Seasonal influences on surface ozone variability in continental South Africa and implications for air quality, Atmos. Chem. Phys., 2018, 18, 15491–15514 CrossRef CAS PubMed.
- V. Vakkari, V.-M. Kerminen, J. P. Beukes, P. Tiitta, P. G. Van Zyl, M. Josipovic, A. D. Venter, K. Jaars, D. R. Worsnop, M. Kulmala and L. Laakso, Rapid changes in biomass burning aerosols by atmospheric oxidation, Geophys. Res. Lett., 2014, 41, 2644–2651 CrossRef CAS.
- K. E. Chiloane, J. P. Beukes, P. G. van Zyl, P. Maritz, V. Vakkari, M. Josipovic, A. D. Venter, K. Jaars, P. Tiitta, M. Kulmala, A. Wiedensohler, C. Liousse, G. V. Mkhatshwa, A. Ramandh and L. Laakso, Spatial, temporal and source contribution assessments of black carbon over the northern interior of South Africa, Atmos. Chem. Phys., 2017, 17, 6177–6196 CrossRef CAS.
-
K. E. Langerman, S. J. Piketh, C. J. Pauw and H. J. Smith, Moving households to cleaner energy through air quality offsets, International Conference on the Domestic Use of Energy (DUE), 2018, pp. 1–8 Search PubMed.
- C. Cohen, M. L. McMorrow, N. A. Martinson, K. Kahn, F. K. Treurnicht, J. Moyes, T. Mkhencele, O. Hellferscee, L. Lebina, M. Moroe, K. Motlhaoleng, F. X. Gómez-Olivé, R. Wagner, S. Tollman, F. Wafawanaka, S. Ngobeni, J. Kleynhans, A. Mathunjwa, A. Buys, L. Maake, N. Wolter, M. Carrim, S. Piketh, B. Language, A. Mathee, A. Von Gottberg and S. Tempia and Group for the PHIRST, Cohort Profile: A Prospective Household Cohort Study of Influenza, Respiratory Syncytial Virus and Other Respiratory Pathogens Community Burden and Transmission Dynamics in South Africa, 2016–2018, Influenza Other Respir. Viruses, 2021, 15, 789 CrossRef CAS PubMed.
- C. Samara, On the redox activity of urban aerosol particles: Implications for size distribution and relationships with organic aerosol components, Atmosphere, 2017, 8, 1–18 CrossRef.
- C. Samara, A. Kouras, K. Kaidoglou, E.-N. Emmanouil-Nikoloussi, C. Simou, M. Bousnaki and A. Kelessis, Ultrastructural alterations in the mouse lung caused by real-life ambient PM10 at Urban Traffic Sites, Sci. Total Environ., 2015, 532, 327–336 CrossRef CAS.
- H. Vreeland, R. Weber, M. Bergin, R. Greenwald, R. Golan, A. G. Russell, V. Verma and J. A. Sarnat, Oxidative potential of PM2.5 during Atlanta rush hour: measurements of in-vehicle dithiothreitol (DTT) activity, Atmos. Environ., 2017, 165, 169–178 CrossRef CAS.
- C. A. Jakober, S. G. Riddle, M. A. Robert, H. Destaillats, M. J. Charles, P. G. Green and M. J. Kleeman, Quinone emissions from gasoline and diesel motor vehicles, Environ. Sci. Technol., 2007, 41, 4548–4554 CrossRef CAS PubMed.
- P. Lin and J. Z. Yu, Generation of reactive oxygen species mediated by humic-like substances in atmospheric aerosols, Environ. Sci. Technol., 2011, 45, 10362–10368 CrossRef CAS.
- G. Uzu, J.-J. Sauvain, A. Baeza-Squiban, M. Riediker, M. S. S. Hohl, S. Val, K. Tack, S. Denys, P. Pradère and C. Dumat, In vitro assessment of the pulmonary toxicity and gastric availability of lead-rich particles from a lead recycling plant, Environ. Sci. Technol., 2011, 45, 7888–7895 CrossRef CAS PubMed.
- A. D. Venter, P. G. Van Zyl, J. P. Beukes, J.-S. Swartz, M. Josipovic, V. Vakkari, L. Laakso and M. Kulmala, Size-resolved characteristics of inorganic ionic species in atmospheric aerosols at a regional background site on the South African Highveld, J. Atmos. Chem., 2018, 75, 285–304 CrossRef CAS.
-
A. D. Venter, Assessment of atmospheric trace metals and water soluble ionic species at two regional background sites, PhD thesis, North-West University (South Africa), Potchefstroom Campus, 2015.
- H. Cachier, M.-P. Bremond and P. Buat-Ménard, Determination of atmospheric soot carbon with a simple thermal method, Tellus B, 1989, 41, 379–390 CrossRef.
- P. C. Mouli, S. V. Mohan, V. Balaram, M. P. Kumar and S. J. Reddy, A study on trace elemental composition of atmospheric aerosols at a semi-arid urban site using ICP-MS technique, Atmos. Environ., 2006, 40, 136–146 CrossRef.
- P. G. Van Zyl, J. P. Beukes, G. du Toit, D. Mabaso, J. Hendriks, V. Vakkari, P. Tiitta, J. J. Pienaar, M. Kulmala and L. Laakso, Assessment of atmospheric trace metals in the Western Bushveld Igneous Complex, South Africa, S. Afr. J. Sci., 2014, 110, 1–10 CrossRef.
- Q. Liu, J. Baumgartner, Y. Zhang, Y. Liu, Y. Sun and M. Zhang, Oxidative potential and inflammatory impacts of source apportioned ambient air pollution in Beijing, Environ. Sci. Technol., 2014, 48, 12920–12929 CrossRef CAS.
- M. Steenhof, I. Gosens, M. Strak, K. J. Godri, G. Hoek, F. R. Cassee, I. S. Mudway, F. J. Kelly, R. M. Harrison, E. Lebret, B. Brunekreef, N. A. H. Janssen and R. H. H. Pieters, In vitro toxicity of particulate matter (PM) collected at different sites in the Netherlands is associated with PM composition, size fraction and oxidative potential - the RAPTES Project, Part. Fibre Toxicol., 2011, 8, 1–15 CrossRef.
- S. Yu, W. Liu, Y. Xu, K. Yi, M. Zhou, S. Tao and W. Liu, Characteristics and oxidative potential of atmospheric PM2.5 in Beijing: Source apportionment and seasonal variation, Sci. Total Environ., 2019, 650(Pt 1), 277–287 CrossRef CAS PubMed.
- D. Chirizzi, D. Cesari, M. R. Guascito, A. Dinoi, L. Giotta, A. Donateo and D. Contini, Influence of Saharan dust outbreaks and carbon content on oxidative potential of water-soluble fractions of PM2.5 and PM10, Atmos. Environ., 2017, 163, 1–8 CrossRef CAS.
- M. G. Perrone, J. Zhou, M. Malandrino, G. Sangiorgi, C. Rizzi, L. Ferrero, J. Dommen and E. Bolzacchini, PM chemical composition and oxidative potential of the soluble fraction of particles at two sites in the urban area of Milan, Northern Italy, Atmos. Environ., 2016, 128, 104–113 CrossRef CAS.
- E. Velali, E. Papachristou, A. Pantazaki, T. Choli-Papadopoulou, S. Planou, A. Kouras, E. Manoli, A. Besis, D. Voutsa and C. Samara, Redox activity and in vitro bioactivity of the water-soluble fraction of urban particulate matter in relation to particle size and chemical composition, Environ. Pollut., 2016, 208, 774–786 CrossRef CAS PubMed.
- F. Shirmohammadi, S. Hasheminassab, D. Wang, J. J. Schauer, M. M. Shafer, R. J. Delfino and C. Sioutas, The relative importance of tailpipe and non-tailpipe emissions on the oxidative potential of ambient particles in Los Angeles, CA, Faraday Discuss., 2016, 189, 361–380 RSC.
- N. A. H. Janssen, A. Yang, M. Strak, M. Steenhof, B. Hellack, M. E. Gerlofs-Nijland, T. Kuhlbusch, F. Kelly, R. Harrison, B. Brunekreef, G. Hoek and F. Cassee, Oxidative potential of particulate matter collected at sites with different source characteristics, Sci. Total Environ., 2014, 472, 572–581 CrossRef CAS PubMed.
- A. Saffari, N. Daher, M. M. Shafer, J. J. Schauer and C. Sioutas, Seasonal and spatial variation in dithiothreitol (DTT) activity of quasi-ultrafine particles in the Los Angeles Basin and its association with chemical species, J. Environ. Sci. Health, Part A, 2014, 49, 441–451 CrossRef CAS PubMed.
- V. Verma, T. Fang, H. Guo, L. King, J. T. Bates, R. E. Peltier, E. Edgerton, A. G. Russell and R. J. Weber, Reactive oxygen species associated with water-soluble PM2.5 in the Southeastern United States: spatiotemporal trends and source apportionment, Atmos. Chem. Phys., 2014, 14, 12915–12930 CrossRef.
- J. G. Charrier and C. Anastasio, On Dithiothreitol (DTT) as a Measure of oxidative potential for ambient particles: Evidence for the importance of soluble transition metals, Atmos. Chem. Phys., 2012, 12, 9321–9333 CrossRef CAS.
- H. A. Jeng, Chemical composition of ambient particulate matter and redox activity, Environ. Monit. Assess., 2010, 169, 597–606 CrossRef CAS PubMed.
- S. Hu, A. Polidori, M. Arhami, M. M. Shafer, J. J. Schauer, A. Cho and C. Sioutas, Redox activity and chemical speciation of size fractioned PM in the communities of the Los Angeles-Long Beach Harbor, Atmos. Chem. Phys., 2008, 8, 6439–6451 CrossRef CAS.
- R. J. Delfino, N. Staimer, T. Tjoa, M. Arhami, A. Polidori, D. L. Gillen, S. C. George, M. M. Shafer, J. J. Schauer and C. Sioutas, Associations of primary and secondary organic aerosols with airway and systemic inflammation in an Elderly Panel Cohort, Epidemiology, 2010, 21(6), 892–902 CrossRef PubMed.
- M. Josipovic, C. Leal-Liousse, B. Crobeddu, A. Baeza-Squiban, C. K. Segakweng, C. Galy-Lacaux, J. P. Beukes, P. G. Van Zyl and G. Fourie, Aerosol characterisation including oxidative potential as a proxy of health impact: A case of a residential site in a highly industrialised area, Clean Air J., 2019, 29, 1–16 Search PubMed.
- F. Yang, C. Liu and H. Qian, Comparison of outdoor and indoor oxidative potential of PM2.5: Pollution levels, temporal patterns, and key constituents, Environ. Int., 2021, 155, 1–9 CrossRef.
- S. S. Khurshid, S. Emmerich and A. Persily, Oxidative potential of particles at a Research House: Influencing factors and comparison with outdoor particles, Build. Environ., 2019, 163, 106275 CrossRef PubMed.
- G. Karavalakis, N. Gysel, D. A. Schmitz, A. K. Cho, C. Sioutas, J. J. Schauer, D. R. Cocker and T. D. Durbin, Impact of biodiesel on regulated and unregulated emissions, and redox and proinflammatory properties of PM emitted from heavy-duty vehicles, Sci. Total Environ., 2017, 584–585, 1230–1238 CrossRef CAS.
- L. Ntziachristos, J. R. Froines, A. K. Cho and C. Sioutas, Relationship between redox activity and chemical speciation of size-fractionated particulate matter, Part. Fibre Toxicol., 2007, 4, 1–12 CrossRef PubMed.
- J. Yang, P. Roth, C. R. Ruehl, M. M. Shafer, D. S. Antkiewicz, T. D. Durbin, D. Cocker, A. Asa-Awuku and G. Karavalakis, Physical, chemical, and toxicological characteristics of particulate emissions from current technology gasoline direct injection vehicles, Sci. Total Environ., 2019, 650, 1182–1194 CrossRef CAS.
- A. Calas, G. Uzu, J.-L. Besombes, J. M. F. Martins, M. Redaelli, S. Weber, A. Charron, A. Albinet, F. Chevrier, G. Brulfert, B. Mesbah, O. Favez and J.-L. Jaffrezo, Seasonal variations and chemical predictors of oxidative potential (OP) of particulate matter (PM), for seven urban French sites, Atmosphere, 2019, 10, 698 CrossRef CAS.
|
This journal is © The Royal Society of Chemistry 2025 |
Click here to see how this site uses Cookies. View our privacy policy here.