DOI:
10.1039/D2RA06545B
(Paper)
RSC Adv., 2022,
12, 33501-33509
Robust acid–base Ln-MOFs: searching for efficient catalysts in cycloaddition of CO2 with epoxides and cascade deacetalization–Knoevenagel reactions†
Received
17th October 2022
, Accepted 17th November 2022
First published on 23rd November 2022
Abstract
A family of microporous and robust Ln(III)-based metal–organic frameworks (1-Ln, Ln = Sm, Eu, Gd, Tb, Dy, Ho, Er, Yb) have been obtained using 4,4′,4′′-nitrilotribenzoic acid (H3NTB) in NMP–HCl solvent. Both single-crystal and powder X-ray diffraction analyses demonstrate that 1-Ln are isostructural and possess 3D frameworks with permanent porosity for Ar and CO2 adsorption. Strikingly, the incorporation of both Lewis acidic lanthanide ions and the basic triphenylamine group into 1-Ln makes them efficient acid–base catalysts for both cycloaddition of epoxides with CO2 and one-pot cascade deacetalization–Knoevenagel reactions. The systematic catalytic studies show that 1-Tb and 1-Yb possess the best catalytic activities for both reactions, indicating the catalytic activities of these Ln-MOFs are strongly dependent on metal Lewis acid sites embedded in the frameworks.
Introduction
Recently, lanthanide based metal–organic frameworks (Ln-MOFs) have been intensely investigated as heterogeneous catalysts because of their unique features, such as inherent porosity, tunable pore sizes, reusability, and a broad range of catalytic sites.1–3 In principle, the active sites of Ln-MOF catalysts can be (i) lanthanide ions with unsaturated coordination environments, (ii) organic or organometallic functional sites realized using pre-designed ligands, and (iii) other catalytic species encapsulated in the pores. The integration of these catalytic sites into one material would promise the Ln-MOFs a powerful platform for heterogeneous catalysis. Currently, Ln-MOFs as Lewis acids have been applied for a number of organic transformations, including cycloaddition of CO2, Knoevenagel condensation, and Heck reactions.4–6
Carbon dioxide (CO2), as the primary greenhouse gas, is also a nontoxic, economic, and renewable C1 building block. CO2 capture and conversion into value-added chemicals are attractive in terms of green chemistry.7,8 Particularly, the cycloaddition of epoxides and CO2 into cyclic carbonates is considered to be one of the most promising approaches for CO2 utilization, given the wide industrial applications of the target cyclic carbonates.9,10 In recent years, it has been reported that some porous Ln-MOFs have shown preeminent catalytic performances on cycloaddition reactions of epoxides and CO2, mainly because these MOFs feature selective CO2 capture and lanthanide ions as Lewis acid sites to activate epoxide.11–15
On the other hand, among various catalytic processes, cascade reactions are sequentially proceeded with two or multiple transformations in one-pot, which eliminate the time-consuming isolation and purification of intermediates.16–18 However, some cascade reactions require the catalysts with antagonistic catalytic sites (e.g., Lewis acidic sites for the first step and basic sites for the second step in deacetalization–Knoevenagel reaction or deacetalization–Henry reaction). Therefore, the catalyst design toward these one-pot cascade reactions is challenging.19–23 In this respect, Ln-MOFs offer the unique possibility of combining isolated Lewis acidic and basic active sites for cascade or cooperative catalysis. To date, several acid–base bifunctional MOFs have shown to be high-performance catalysts for one-pot cascade reactions.4,11,24–26
The Knoevenagel reaction as one important synthetic method for the C–C bond formation, is typically catalyzed by base,27 and triphenylamine groups in a MOF as a weak base for the Knoevenagel reaction have been reported by Duan's and Wen's group.28,29 Meanwhile, the lanthanide ions embedded in Ln-MOFs have proven to be valuable Lewis acid sites for a number of organic reactions, as mentioned before.4–6 Bearing these considerations in mind, one would expect a Ln-MOF incorporating triphenylamine group to be an acid–base bifunctional catalyst for both cycloaddition of epoxides with CO2 and one-pot deacetalization–Knoevenagel reaction.
In this work, using 4,4′,4′′-nitrilotribenzoic acid (H3NTB, a typical triphenylamine ligand) and lanthanide nitrates dissolved in NMP–HCl mixture, we have solvothermally synthesized a new family of 3D microporous Ln-MOFs (denoted 1-Ln, Ln = Sm, Eu, Gd, Tb, Dy, Ho, Er, Yb). The structural analyses reveal 1-Ln are intrinsically isostructural, which offer the opportunity to determine the effect of the metal center on catalytic performance. All 1-Ln solids exhibit remarkable robustness and permanent porosity evaluated by Ar adsorption analysis. Their CO2 adsorption at 273, 298 K/1 bar are also investigated. Interestingly, the incorporation of both Lewis acidic lanthanide ions and basic triphenylamine group into one single framework makes them highly efficient catalysts for both cycloaddition of epoxides with CO2 and one-pot cascade deacetalization–Knoevenagel condensation reactions. Among them, 1-Tb and 1-Yb possess the best catalytic activities for both two reactions. More strikingly, the systematic catalytic studies reveal that the catalytic activities of these Ln-MOFs are strongly dependent on Lewis acidic lanthanide ions embedded in the framework, uncovering the possibilities to develop Tb- and Yb-MOFs as high-performance Lewis acid catalysts.
Results and discussion
Structure analyses of 1-Ln crystals
Solvothermal reactions of different lanthanide salts with H3NTB in NMP–HCl mixture harvest a family of crystalline Ln-MOFs, 1-Ln (Ln = Sm, Eu, Gd, Tb, Dy, Ho, Er, Yb), which are isostructural as checked by powder X-ray diffraction (XRD, Fig. 2) and infra-red spectroscopy (Fig. S3†). Under the same synthetic conditions, LaCl3·6H2O affords a different structure that would be reported in due course. Single crystal X-ray diffraction (SCXRD) analyses of 1-Tb and 1-Yb show that they crystallize in the triclinic space group P
(Table S1†) and have the formula of {[Ln(NTB)(NMP)]·(NMP)}n, which are in good accordance with their TG analyses (Fig. S4†). SCXRD analyses reveal 1-Ln have a porous architecture (Fig. 1 and 2). Therefore, only the structure of 1-Yb is briefly discussed herein. The asymmetric unit of 1-Yb contains one Yb3+ cation, one NTB3− ligand, one coordinated and one free guest NMP molecule (Fig. S1†). As shown in Fig. 1a, each Yb3+ is coordinated by seven oxygen atoms from one NMP molecule and six μ2-η1:η1 carboxylate groups that belong to six different ligands. Interestingly, every two Yb3+ ions are four-fold bridged by four μ2-η1:η1 carboxylate groups to form a dimeric unit. These dimeric units are further two-fold bridged by another two μ2-η1:η1 carboxylate groups to generate a 1D Yb-COO inorganic chains running along [100] (Fig. 1b). These Yb-COO inorganic chains are connected by NTB3− ligands to define the three-dimensional porous framework (Fig. 2). The framework possesses two kinds of rhombus channels, one with diagonals of 7.1 × 5.9 Å2 (measured between the opposite atoms of N⋯N and Yb⋯Yb, and considering the van der Waals radii) along [100] which are aligned by coordinated NMP molecules, and the other one with diagonals of 8.8 × 5.8 Å2 (measured between the opposite atoms of N⋯N and Yb⋯Yb) along [110] which are filled by guest NMP molecules. After removal of all NMP molecules, the free void calculated by PLATON is up to 47.4% of the crystal volume. Thus, 1-Yb is a highly microporous framework, which would be suitable for gas adsorption. Moreover, the incorporation of Lewis acidic lanthanide centers and Lewis basic triphenylamine sites in the channels indicates that 1-Yb could be a potential acid–base heterogeneous catalyst. With these structures (1-Sm, Eu, Gd, Tb, Dy, Ho, Er, Yb) in hand, comparative experiments were performed to determine the effect of the metal centers on catalytic performance and to search for more efficient catalysts.
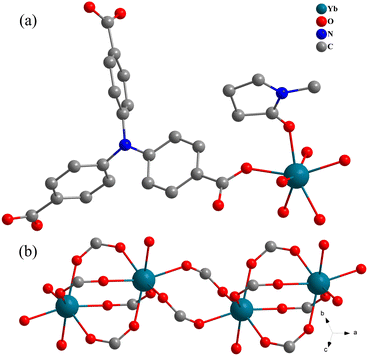 |
| Fig. 1 (a) The coordination environment around Yb3+ in the 1-Yb; (b) 1D Yb-COO inorganic chain. | |
 |
| Fig. 2 (a) 3D porous framework of 1-Yb showing channels along [100], with removal of all coordinated and guest NMP molecules for clarity; (b) perspective view of channels along [110], with NMP guest molecules occupying the channels. | |
PXRD analyses, thermal stability of the frameworks, Ar and CO2 adsorption isotherms
The PXRD profiles of as-synthesized 1-Ln solids match well with their simulated ones, verifying the phase purity of the as-synthesized bulk samples (Fig. S2†). Furthermore, all 1-Ln solids display similar and excellent thermal stability (Fig. S4†). As shown by thermogravimetric analyzer (TGA), the guest-free phases of 1-Ln are thermally stable up to 500 °C. This exceptional robustness of the frameworks is also observed in other Ln-MOFs structurally built on Ln-COO inorganic chains.30–33
Ar adsorption experiments were performed at 87 K to assess the permanent porosity of all compounds. The adsorption isotherms (Fig. 3 and S5†) demonstrate type-I adsorption which is typical of microporous materials, with the BET (Brunauer–Emmett–Teller) surface area of 113.7 (1-Sm), 128.1 (1-Eu), 222.0 (1-Gd), 299.0 (1-Tb), 268.8 (1-Dy), 261.2 (1-Ho), 274.7 (1-Er), and 300.7 (1-Yb) m2 g−1, respectively. The total pore volumes are 0.105 (1-Sm), 0.111 (1-Eu), 0.152 (1-Gd), 0.204 (1-Tb), 0.176 (2-Dy), 0.182 (1-Ho), 0.202 (1-Er), and 0.209 (1-Yb) cm3 g−1, respectively. These values are comparable to other porous lanthanide MOFs.33–35
Encouraged by the robustness and permanent porosity of 1-Ln, we investigated their CO2 adsorption behaviors. It is clearly demonstrated that all 1-Ln solids are readily accessible to CO2 (Fig. S5†). For 1-Tb and 1-Yb, their CO2 adsorption isotherms at 273 and 298 K are shown in Fig. 3. The maximal CO2 capacities is 27.5, 15.9 cm3 g−1 (for 1-Tb) and 29.4, 16.5 cm3 g−1 (for 1-Yb) respectively. In order to gain more insights into the framework–CO2 interactions, adsorption heat of CO2 (Qst) was calculated by using the viral equation based on the isotherms at 273 K and 298 K. As shown in Fig. S6,† the Qst value at zero coverage is around 22.5 kJ mol−1 (for 1-Tb) and 25.0 kJ mol−1 (for 1-Yb), respectively, which are comparable to some MOFs such as [Zn2(L)(dpe)2](NO3)·(DMF)3·(H2O)2 (20.5 kJ mol−1; L = 1,3-bis(3,5-dicarboxyphenyl)imidazolium, dpe = 1,2-di(4-pyridyl)ethylene)36 and FJU-14-BF4 (18.8 kJ mol−1),37 indicating moderate interactions between the framework and guest CO2 molecules.
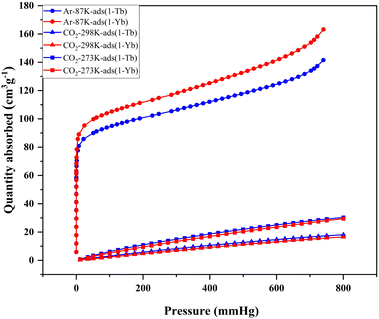 |
| Fig. 3 The Ar adsorption isotherms at 87 K and the CO2 adsorption isotherms at 273 K and 298 K of 1-Tb and 1-Yb. | |
Catalytic cycloaddition of CO2 and epoxides
On account of the considerable CO2 adsorption quantity, the accessible channels, and the coexisting Lewis acid–base sites, the catalytic performances of 1-Ln towards the cycloaddition of CO2 and epoxides into cyclic carbonates are investigated. We wonder the effect of the metal center on catalytic performance among the eight Ln-MOF candidates (1-Sm, Eu, Gd, Tb, Dy, Ho, Er, Yb). Firstly, with 1-Yb and n-Bu4NBr (tetra-n-tertbutylammonium bromide) as catalytic system, the cycloaddition between CO2 and epichlorohydrin under ambient temperature has been used as a model reaction to screen the optimal conditions. After detailed investigations, the optimal conditions are set as follows: epichlorohydrin (4 mmol), CO2 (1 atm), 1-Yb catalyst (0.02 mmol based on Ln metal sites; 0.5 mol%) and cocatalyst n-Bu4NBr (0.2 mmol; 5 mol%), room temperature, 36 hours. As seen in Table 1, in the case of the reaction without any catalyst (entry 1), the reaction does not proceed. Without cocatalyst n-Bu4NBr, pure 1-Yb catalyst shows a low conversion of 12.0% (entry 2); while without 1-Yb, n-Bu4NBr alone lead to almost no reactivity (4.8%, entry 3). However, when 1-Yb catalyst and cocatalyst n-Bu4NBr were added as a binary catalytic system (entry 4), the conversion of epichlorohydrin is up to 97%, highlighting the remarkable synergistic effect of the binary catalytic system.
Table 1 Cycloaddition of CO2 and epichlorohydrin with different 1-Ln catalystsa
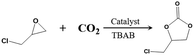
|
Entry |
Catalyst |
Catalyst (mol%) |
n-Bu4NBr (mol%) |
Yieldb (%) |
Reaction conditions: 4 mmol of epichlorohydrin, solvent free, CO2 (1 atm). The yields were determined by 1H NMR spectrum of the crude reaction mixtures. |
1 |
— |
0 |
0 |
<1.0 |
2 |
1-Yb |
0.5 |
0 |
12.0 |
3 |
— |
0 |
5 |
4.8 |
4 |
1-Yb |
0.5 |
5 |
97.0 |
5 |
1-Sm |
0.5 |
5 |
89.0 |
6 |
1-Eu |
0.5 |
5 |
83.0 |
7 |
1-Gd |
0.5 |
5 |
95.0 |
8 |
1-Tb |
0.5 |
5 |
99.0 |
8 |
1-Dy |
0.5 |
5 |
79.0 |
9 |
1-Ho |
0.5 |
5 |
81.0 |
10 |
1-Er |
0.5 |
5 |
87.0 |
Then using the optimized conditions, we extend this reaction to other seven isomorphic MOF candidates to search for efficient catalysts and determine the effect of the metal centers on catalytic performances. As illustrated in Table 1, the comparative catalytic results reveal that 1-Ln solids are all valuable catalyst candidates for cycloaddition reaction of CO2 and epoxides. Among them, 1-Gd, Tb and Yb demonstrate the highest catalytic activity, comparable to the most effective MOF catalysts reported in literature (see Table S3†). Fig. 4 demonstrates the relationship between the catalytic yield of each Ln-MOFs and atomic number of Ln (ZLn). It is found that the catalytic yield shows two increase stages, one with a gradual increase from Sm (89%) to Tb (99%) and then an abrupt decrease at Dy (79%), and the other one with gradual increase from Dy to Yb (97%).
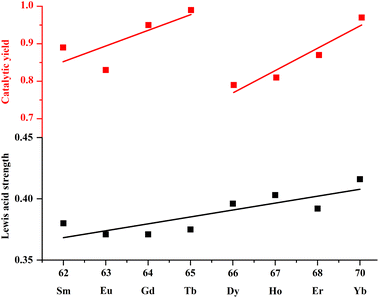 |
| Fig. 4 The trend chart showing catalytic yield (activity) of Ln-MOFs vs. ZLn in catalyzing CO2-cycloaddition reaction and the Lewis acid strength of Ln3+ vs. ZLn. | |
Generally, the strength of a Lewis acid is a critical factor in determining its catalytic activity, because a certain threshold of Lewis acidity is required to induce catalytic chemical transformations. In Fig. 4, we also plot the empirical scale for Lewis acid strength of Ln3+ along with ZLn based on the reported data, showing an almost linear increase of Lewis acid strength of Ln3+ against ZLn; the heavier Ln3+, the more Lewis acidic.38 Obviously, the trend of the catalytic yield (activity) of Ln-MOFs vs. ZLn does not coincide with that of the Lewis acid strength vs. ZLn. These analyses demonstrate that the catalytic activity of Ln-MOFs, at least for 1-Tb, is not solely dependent on Lewis acid strength of Ln3+, but also on some other factors. We tend to presume that, compared to Yb3+, the lighter lanthanide ions like Tb3+ possesses high and variable coordination number allowing easy rearrangements for substrate activation.39,40 Therefore, we attribute the anomalously high activity of 1-Tb to the combining effect of the modest Lewis acid strength and the labile coordination environment of Tb3+ during the catalysis. Note that the underlying mechanic path of 1-Tb and 1-Yb may be different, and new systems or experiments should be designed to elucidate the exact catalytic mechanism in the future. Importantly, the present study not only confirms the available Lewis acidity of Ln3+ ions in MOFs, but also point out the way to develop Tb- and Yb-MOFs as high-performance Lewis acid catalysts.
Lastly, with the optimal catalysts in hand, we turn our attention to the recyclability, stability, and catalytic generality of 1-Yb as a heterogeneous catalyst. 1-Yb can be readily recovered, washed thoroughly with CH2Cl2, and used for next runs. Within five catalytic runs, high yields of 97–99% are maintained (Fig. S7†), suggesting that 1-Yb possessed high recyclability to the cycloaddition reaction. In addition, a PXRD pattern of 1-Yb recovered from the fifth run is consistent with the one of the as-synthesized, confirming the structural integrity of 1-Yb during the catalytic process (Fig. S8†). Furthermore, the catalytic generality over 1-Yb can be extended to several typical epoxide substrates with product yields from moderate to good (Table S2†). These results show that stability and heterogeneous nature of the 1-Yb catalyst. Based on structural analysis and catalytic performances of 1-Yb, a tentative synergistic catalytic mechanism for the cycloaddition of CO2 and epoxides over 1-Yb/n-Bu4NBr is proposed in Fig. S9.† First, dissociation of coordinated NMP of 1-Yb occurs and the open Lewis Yb active site coordinates with oxygen atom of the epoxide. This coordination bond polarizes the C–O bond to activate the epoxide. The activated epoxide is opened by Br− ion of TBAB to generate a metal-ordinated bromoalkoxide intermediate. Next, CO2 was nucleophilically attacked by the O atom of bromoalkoxide to form a metal carbonate, which is finally converted to cyclic carbonate by intramolecular (SN2-type nucleophilic) ring-closure reaction, regenerating the catalyst and co-catalyst.10
Catalytic deacetalization–Knoevenagel reactions
Owing to the incorporation of both lanthanide Lewis acidic sites and triphenylamine Lewis basic sites within their porous frameworks, 1-Ln could be viewed as a kind of potential bifunctional acid–base catalysts. In order to prove this point, 1-Ln crystals were used to study their catalytic activities on one-pot cascade deacetalization–Knoevenagel reactions. The reaction between benzaldehyde dimethylacetal (BD) and malononitrile (MA) under solvent-free condition was taken as the template reaction to investigate the catalytic activities of these Ln-MOF solids (Table 2). At the first step of the catalysis, Ln ions as a Lewis acid can catalyze the deprotection of BD to give benzaldehyde (BA), and at the second step, triphenylamine as a weak Lewis base can catalyze Knoevenagel condensation reaction between BA and MA to afford benzylidene malononitrile (BM).
Table 2 One-pot cascade deacetalization–Knoevenagel reaction between benzaldehyde dimethylacetal (BD) and malononitrile (MA) catalyzed by different 1-Ln catalystsa
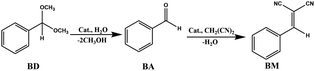
|
Entry |
Catalyst |
Conversion of BDb (%) |
Conversion of BAc (%) |
Yield of BMb (%) |
Reaction conditions: BD (1 mmol), H2O (3 mmol), MA (2 mmol), catalysts (0.5 mol%), 60 °C, 6 h, N2 atmosphere. The yields were determined by 1H NMR spectrum of the crude reaction mixtures. The conversion of intermediate BA is defined by the ratio of (yield of BM)/(conversion of BD). Control experiment was performed without water in the presence of 1-Yb. Control experiment was done without 1-Yb in the presence of water. |
1d |
1-Yb |
0 |
0 |
0 |
2e |
— |
0 |
0 |
0 |
3 |
1-Sm |
83 |
81 |
68 |
4 |
1-Eu |
93 |
83 |
78 |
5 |
1-Gd |
96 |
96 |
93 |
6 |
1-Tb |
98 |
100 |
98 |
7 |
1-Dy |
79 |
98 |
77 |
8 |
1-Ho |
88 |
96 |
84 |
9 |
1-Er |
86 |
97 |
86 |
10 |
1-Yb |
97 |
100 |
97 |
The catalytic reactions were performed using the following conditions: BD (1 mmol), H2O (3 mmol), MA (2 mmol), catalysts (0.5 mol%), 60 °C, 6 h, N2 atmosphere. Experimental results are given in Table 2, showing that 1-Ln solids are all valuable catalyst candidates for one-pot cascade reaction. Among them, 1-Tb and 1-Yb demonstrate the highest catalytic activity. Control experiments were also performed to figure out the role of components during the catalytic procedure. H2O was necessary in the deacetalization (step I) process, as the reaction was sluggish without water even in the presence of 1-Yb (entry 1). As shown in entry 2, the reaction did not proceed without 1-Yb.
In order to clarify the active catalytic site for each step, we calculate and plot the conversion of BD and intermediate BA, and the yield of BM against ZLn (the coordination number of Ln) in Table 2 and Fig. 5. The conversion of BD to BA in first step (Fig. 5a) clearly show that all Ln3+ cations in MOFs have the Lewis acidity enough to efficiently catalyze the deprotection of BD, with two maxima at 1-Tb (98%), 1-Yb (97%) and two minima at 1-Sm (83%) and 1-Dy (79%). At the first step of the one-pot catalysis, the trend of the catalytic activity in Ln-MOFs is not regularly increased along with ZLn but very similar to that found in catalytic cycloaddition of CO2 and epoxides, demonstrating Ln3+ cations embedded in MOF as Lewis acids is distinct from homogeneous species, possible due to their unique coordination environment in MOF solid. In second step, the conversion of intermediate BA to the product BM, which is defined by the ratio of (yield of BM)/(conversion of BD), is increased from 81% for Sm to almost 100% for Gd, Tb, Dy, Ho, Er, Yb (Fig. 5b). Generally, Knoevenagel condensation reactions are considered to be base-catalyzed.27–29 However, for comparison, control experiments show that the conversion of BA to BM catalyzed by sole triphenylamine or H3NTB is only 70 or 46%, respectively. The present study confirms that Ln3+ especially for Tb3+, Yb3+ in MOFs plays an important role in accelerating the Knoevenagel reaction. This synergistic effect of the Lewis acidic metal centers and Lewis base sites in porous MOFs on catalyzing Knoevenagel reaction is also observed in other scientific studies.6,29,31,41
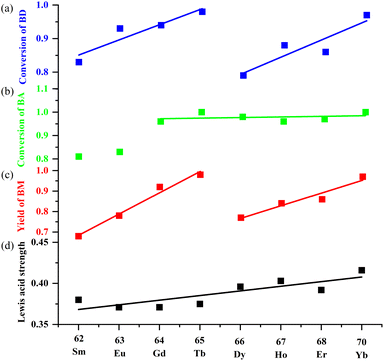 |
| Fig. 5 The trend chart showing (a) the conversion of BD, (b) the conversion of intermediate BA, and (c) the yield of BM vs. ZLn (the coordination number of Ln) in catalyzing one-pot cascade reaction and (d) the Lewis acid strength of Ln3+ vs. ZLn. | |
The above analyses reveal that both steps are strongly correlating with the catalytic activity of lanthanide ions embedded in the framework. As a consequence, the total yields of BM show 2-Tb and 2-Yb have the highest catalytic activity towards one-pot reaction. Interestingly, similar to that found in Fig. 4, the trend of catalytic activity of each Ln-MOFs vs. ZLn (Fig. 5c) also shows two increase stages, one with a gradual increase from Sm (68%) to Tb (98%) and then an abrupt decrease at Dy (77%), and the other one with gradual increase from Dy (77%) to Yb (97%). Once again, the present study confirm the feasibility to develop Tb- and Yb-MOFs as high-performance Lewis acid catalysts, comparable to the most effective MOF catalysts reported in literature (see Table S5†).
Finally, we investigate the recyclability, stability, and catalytic generality of 1-Yb. After five consecutive catalytic runs under optimized conditions, 1-Yb kept high catalytic activity (Fig. S10†). Moreover, the PXRD patterns between the recovered and the original one are almost identical, confirming the structural integrity of 1-Yb (Fig. S11†). The recovered 1-Yb was also evaluated by Ar adsorption, showing the retention of the porous framework (Fig. S12†). In addition, 1-Yb had the general high catalytic activity on a series of epoxides with various substitutes under the optimal reaction conditions (Table S4†). All results show the stability and heterogeneous nature of the 1-Yb catalyst. Based on the structure and catalytic behaviors of 1-Yb, a possible catalytic mechanism for one-pot cascade deacetalization–Knoevenagel reaction on 1-Yb is proposed in Fig. S13.†
Conclusions
In summary, we have synthesized a family of robust and microporous Ln-MOFs (1-Ln, Ln = Sm, Eu, Gd, Tb, Dy, Ho, Er, Yb) based on H3NTB, demonstrating permanent porosity for Ar and CO2 adsorption abilities. Importantly, the combination of Lewis acidic lanthanide ions and basic triphenylamine group into the framework of 1-Ln makes them efficient acid–base catalysts for both cycloaddition of CO2 with epoxides and one-pot cascade deacetalization–Knoevenagel reactions. Among them, 1-Tb and 1-Yb demonstrates the best catalytic activities for both two reactions. More strikingly, the systematic catalytic studies reveal that the catalytic activities of these Ln-MOFs are strongly dependent on Lewis acidic lanthanide ions embedded in the framework, opening up the possibilities to develop Tb- and Yb-MOFs as high-performance Lewis acid catalysts. Work is underway to synthesize new Ln-MOFs in our lab.
Experimental
Materials and instruments
Materials and instruments can be seen in ESI S1† part.
Synthesis
Synthesis of {[Sm(NTB)(NMP)]·(NMP)}n (1-Sm). A mixture of Sm(NO3)3·6H2O (16.0 mg), H3NTB (23.3 mg), NMP (1.0 mL), HCl (0.01 M, 0.2 mL), H2O (0.3 mL) was sealed in a 23.0 mL Teflon-lined stainless-steel container, which was heated at 85 °C for 21 hours to afford block crystals. The collected crystals were dried in air at room temperature. Yield: 11.24 mg. IR/cm−1 (KBr): 3424 (br), 3045 (w), 2925 (w), 2873 (w), 1687 (m), 1627 (m), 1584 (m), 1534 (m), 1393 (m), 1332 (m), 1178 (m), 1144 (w), 1117 (w), 1015 (w), 925 (w), 831 (m), 783 (s), 719 (m), 659 (w), 628 (w), 580 (m), 529 (w), 506 (m), 470 (w), 441 (w).
Synthesis of {[Eu(NTB)(NMP)]·(NMP)}n (1-Eu). 1-Eu was synthesized by using the same procedure except by using Eu(NO3)3·6H2O (16.6 mg). Yield: 9.51 mg. IR/cm−1 (KBr): 3430 (br), 3044 (w), 2926 (w), 2873 (w), 1687 (m), 1628 (m), 1585 (m), 1535 (m), 1392 (s), 1332 (m), 1262 (w), 1177 (m), 1144 (w), 1015 (w), 831 (m), 784 (s), 719 (m), 659 (w), 628 (w), 559 (m), 529 (w), 506 (m), 470 (w), 441 (w).
Synthesis of {[Gd(NTB)(NMP)]·(NMP)}n (1-Gd). 1-Gd was synthesized by using the same procedure except by using Gd(NO3)3·6H2O (14.5 mg). Yield: 11.49 mg. IR/cm−1 (KBr): 3393 (br), 2926 (w), 1686 (w), 1655 (w), 1625 (w), 1585 (w), 1535 (w), 1401 (s), 1315 (w), 1275 (w), 1170 (m), 1106 (w), 1014 (w), 924 (m), 582 (m), 784 (s), 710 (m), 659 (w), 628 (w), 565 (m), 525 (w), 505 (m), 470 (w), 442 (w).
Synthesis of {[Tb(NTB)(NMP)]·(NMP)}n (1-Tb). 1-Tb was synthesized by using the same procedure except by using Tb(NO3)3·6H2O (27.2 mg). Yield: 25.65 mg. IR/cm−1 (KBr): 3319 (br), 1654 (m), 1596 (m), 1532 (m), 1401 (s), 1316 (w), 1281 (w), 1176 (m), 1150 (w), 1105 (w), 1013 (m), 954 (m), 859 (m), 783 (s), 710 (m), 672 (w), 628 (w), 568 (m), 521 (w),496 (w), 438 (w).
Synthesis of {[Dy(NTB)(NMP)]·(NMP)}n (1-Dy). 1-Dy was synthesized by using the same procedure except by using Dy(NO3)3·6H2O (18.3 mg). Yield: 19.97 mg. IR/cm−1 (KBr): 3434 (br), 3044 (w), 2916 (w), 2873 (w), 1687 (m), 1630 (m), 1585 (m), 1537 (w), 1393 (s), 1331 (w), 1307 (w), 1260 (w), 1178 (w), 1144 (w), 1116 (w), 1015 (w), 865 (w), 831 (w), 783 (s), 719 (w), 659 (w), 628 (w), 584 (w), 561 (w), 530 (w), 506 (w), 470 (w), 442 (w).
Synthesis of {[Ho(NTB)(NMP)]·(NMP)}n (1-Ho). 1-Ho was synthesized by using the same procedure except by using Ho(NO3)3·6H2O (17.6 mg). Yield: 20.51 mg. IR/cm−1 (KBr): 3428 (br), 3044 (w), 2925 (w), 2874 (w), 1687 (m), 1632 (m), 1586 (m), 1539 (m), 1514 (w), 1401 (s), 1331 (m), 1261 (w), 1178 (w), 1145 (w), 1117 (w), 1105 (w), 866 (w), 832 (m), 784 (s), 719 (m), 659 (m), 628 (w), 585 (m), 563 (w), 531 (w), 507 (w), 470 (w), 443 (w).
Synthesis of {[Er(NTB)(NMP)]·(NMP) }n (1-Er). 1-Er was synthesized by using the same procedure except by using Er(NO3)3·6H2O (30.0 mg). Yield: 21.32 mg. IR/cm−1 (KBr): 3424 (br), 3044 (w), 2919 (w), 2873 (w), 1687 (m), 1632 (m), 1586 (m), 1539 (m), 1512 (w), 1401 (s), 1307 (m), 1261 (w), 1178 (w), 1145 (w), 1117 (w), 1015 (w), 866 (w), 832 (m), 784 (s), 719 (m), 659 (m), 627 (w), 585 (m), 563 (w), 531 (w), 507 (w), 470 (w), 442 (w).
Synthesis of {[Yb(NTB)(NMP)]·(NMP)}n (1-Yb). 1-Yb was synthesized by using the same procedure except by using YbCl3·6H2O (15.5 mg). Yield: 9.62 mg. IR/cm−1 (KBr): 3415 (br), 3044 (w), 2921 (w), 2874 (w), 1687 (m), 1633 (m), 1588 (m), 1540 (m), 1512 (w), 1401 (s), 1331 (m), 1261 (w), 1178 (w), 1145 (w), 1117 (w), 1105 (w), 925 (w), 866 (w), 833 (w), 784 (s), 719 (m), 659 (m), 628 (w), 588 (m), 565 (w), 531 (w), 509 (w), 471 (w), 443 (w).
Single-crystal structure characterization of the 1-Ln crystals
SCXRD data for 1-Tb and 1-Yb were performed on a Rigaku Oxford X-ray Diffractometer with graphite-monochromated MoKα radiation (λ = 0.71073 Å) at 293 K. All absorption corrections were applied using multi-scan technique. The crystal structures of 1-Ln were solved by the direct method and refined through full-matrix least-squares techniques method on F2 using the Olex2 crystallographic software package.42,43 Full crystallographic data for 1-Tb and 1-Yb has been deposited with the CCDC 2210635 and 2210636. The crystallographic data for 1-Tb and 1-Yb are summarized in Table S1.†
Ar and CO2 adsorption isotherms
Ar adsorption isotherm was recorded at 87 K on a micromeritics ASAP2460 analyzer. The sample was activated under vacuum by heating at 200 °C overnight. The specific surface area was calculated from the data in the adsorption branch at p/p0 = 0.05–0.30. The total pore volume was calculated from the uptake at p/p0 of 0.950. CO2 adsorption isotherms was performed at 273 and 298 K respectively.
General procedure for cycloaddition of CO2 and epoxides
In a typical catalytic reaction, epichlorohydrin (4 mmol) and cocatalyst n-Bu4NBr (0.2 mol; 5 mol%) were added to the Schlenk tube, to which was further added 1-Ln (Ln = Sm, Eu, Gd, Tb, Dy, Ho, Er, Yb) (2 mol% based on metal ion), and then slowly stirred under 1 atm CO2 pressure for 36 hours at room temperature.
General procedure for one-pot cascade deacetalization–Knoevenagel reactions
In a typical catalytic reaction, benzaldehyde dimethylacetal (1 mmol), H2O (3 mmol) and malononitrile (2 mmol) and were added to the Schlenk tube, to which was further added 1-Ln (Ln = Sm, Eu, Gd, Tb, Dy, Ho, Er, Yb) (2 mol% based on Ln3+), and then slowly stirred for 6 hours at 60 °C in a nitrogen atmosphere.
Author contributions
Qingxia Yao, Yi Qiu, Jun Li: project administration, supervision. Xuezhen Si, Qingxia Yao: writing original draft, methodology, data collection and analysis. Xuze Pan, Jintang Xue, Minglei Cao, Wenzeng Duan, Xianqiang Huang: data collection and analysis. Yi Qiu, Jie Su: X-ray single-crystal structural analysis.
Conflicts of interest
There are no conflicts to declare.
Acknowledgements
The work was financially supported by the Natural Science Foundation of Shandong Province (No. ZR2022MB137 and ZR2019MB043), the National Natural Science Foundation of China (22003003, 21871125, and 21501086). Yao Q. thanks the financial supports from Liaocheng University (319260119, HFC202119) and the Scientific Research Foundation for the Returned Overseas Chinese Scholars, State Education Ministry.
Notes and references
- Ć. Pagis, M. Ferbinteanu, G. Rothenberg and S. Tanase, Lanthanide-based metal organic frameworks: synthetic strategies and catalytic applications, ACS Catal., 2016, 6(9), 6063–6072 CrossRef.
- X. Shi, B. Cao, J. Liu, J. Zhang and Y. Du, Rare-earth-based metal–organic frameworks as multifunctional platforms for catalytic conversion, Small, 2021, 17, 2005371 CrossRef CAS PubMed.
- Y. Zhang, S. Liu, Z.-S. Zhao, Z. Wang, R. Zhang, L. Li and Z.-B. Han, Recent progress in lanthanide metal–organic frameworks and their derivatives in catalytic applications, Inorg. Chem. Front., 2021, 8, 590–619 RSC.
- H. Chen, S. Liu, H. Lv, Q.-P. Qin and X. Zhang, Nanoporous {Y2}–organic frameworks for excellent catalytic performance on the cycloaddition reaction of epoxides with CO2 and deacetalization–Knoevenagel condensation, ACS Appl. Mater. Interfaces, 2022, 14, 18589–18599 CrossRef CAS PubMed.
- G.-Q. Huang, J. Chen, Y.-L. Huang, K. Wu, D. Luo, J. K. Jin, J. Zheng, S.-H. Xu and W. Lu, Mixed-linker isoreticular Zn(II) metal–organic frameworks as brønsted acid–base bifunctional catalysts for Knoevenagel condensation reactions, Inorg. Chem., 2022, 61, 8339–8348 CrossRef CAS PubMed.
- T. Zhang, H. Chen, S. Liu, H. Lv, X. Zhang and Q. Li, Highly Robust {Ln4}–organic frameworks (Ln = Ho, Yb) for excellent catalytic performance on cycloaddition reaction of epoxides with CO2 and Knoevenagel condensation, ACS Catal., 2021, 11, 14916–14925 CrossRef CAS.
- T. Sakakura, J.-C. Choi and H. Yasuda, Transformation of carbon dioxide, Chem. Rev., 2007, 107, 2365–2387 CrossRef CAS PubMed.
- P. Nugent, Y. Belmabkhout, S. D. Burd, A. J. Cairns, R. Luebke, K. Forrest, T. Pham, S. Ma, B. Space, L. Wojtas, M. Eddaoudi and M. J. Zaworotko, Porous materials with optimal adsorption thermodynamics and kinetics for CO2 separation, Nature, 2013, 495, 80–84 CrossRef CAS PubMed.
- F. Zhang, Y. Wang, X. Zhang, X. Zhang, H. Liu and B. Han, Recent advances in the coupling of CO2 and epoxides into cyclic carbonates under halogen-free condition, Green Chem. Eng., 2020, 1, 82–93 CrossRef.
- X. Huang, X. Gu, H. Zhang, G. Shen, S. Gong, B. Yang, Y. Wang and Y. Chen, Decavanadate-based clusters as bifunctional catalysts for efficient treatment of carbon dioxide and simulant sulfur mustard, J. CO2 Util., 2021, 45, 101419 CrossRef CAS.
- H. Chen, Z. Zhang, T. Hu and X. Zhang, Nanochannel {InZn}–organic framework with a high catalytic performance on CO2 chemical fixation and deacetalization–Knoevenagel condensation, Inorg. Chem., 2021, 60, 16429–16438 CrossRef CAS PubMed.
- L. Zhang, S. Yuan, L. Feng, B. Guo, J.-S. Qin, B. Xu, C. Lollar, D. Sun and H.-C. Zhou, Pore-environment engineering with multiple metal sites in rare-earth porphyrinic metal–organic frameworks, Angew. Chem., Int. Ed., 2018, 57, 5095–5099 CrossRef CAS PubMed.
- V. Guillerm, Ł. J. Weseliński, Y. Belmabkhout, A. Cairns, V. D'Elia, Ł. Wojtas, K. Adil and M. Eddaoudi, Discovery and introduction of a (3,18)-connected net as an ideal blueprint for the design of metal–organic frameworks, Nat. Chem., 2014, 6, 673–680 CrossRef CAS PubMed.
- N. Wei, R.-X. Zuo, Y.-Y. Zhang, Z.-B. Han and X.-J. Gu, Robust high-connected rare-earth MOFs as efficient heterogeneous catalysts for CO2 conversion, Chem. Commun., 2017, 53, 3224–3227 RSC.
- N. Wei, Y. Zhang, L. Liu, Z.-B. Han and D.-Q. Yuan, Pentanuclear Yb(III) cluster-based metal–organic frameworks as heterogeneous catalysts for CO2 conversion, Appl. Catal., B, 2017, 219, 603–610 CrossRef CAS.
- H. Chen, Z. Yang, H. Peng, K. Jie, P. Li, S. Ding, W. Guo, X. Suo, J. Liu, R. Yan, W. Liu, C. L. Do-Thanh, H. Wang, Z. Wang, L. Han, W. Yang and S. Dai, A bifunctional zeolitic porous liquid with incompatible Lewis pairs for antagonistic cascade catalysis, Chem, 2021, 7, 3340–3358 CAS.
- K. C. Nicolaou, D. J. Edmonds and P. G. Bulger, Cascade reactions in total synthesis, Angew. Chem., Int. Ed., 2006, 45, 7134–7186 CrossRef CAS PubMed.
- K. C. Nicolaou and J. S. Chen, The art of total synthesis through cascade reactions, Chem. Soc. Rev., 2009, 38, 2993–3009 RSC.
- Z. Jia, K. Wang, B. Tan and Y. Gu, Hollow hyper-cross-linked nanospheres with acid and base sites as efficient and water-stable catalysts for one-pot tandem reactions, ACS Catal., 2017, 7, 3693–3702 CrossRef CAS.
- A. Gaona, U. Díaz and A. Corma, Functional acid and base hybrid catalysts organized by associated (organo) aluminosilicate layers for C–C bond forming reactions and tandem processes, Chem. Mater., 2017, 29, 1599–1612 CrossRef CAS.
- M. A. Isaacs, C. M. A. Parlett, N. Robinson, L. J. Durndell, J. C. Manayil, S. K. Beaumont, S. Jiang, N. S. Hondow, A. C. Lamb and D. Jampaiah, et al., A spatially orthogonal hierarchically porous acid–base catalyst for cascade and antagonistic reactions, Nat. Catal., 2020, 3, 921 CrossRef CAS.
- L.-C. Lee, J. Lu, M. Weck and C. W. Jones, Acid–base bifunctional shell cross-linked micelle nanoreactor for one-pot tandem reaction, ACS Catal., 2016, 6, 784–787 CrossRef CAS.
- K. Motokura, M. Tada and Y. Iwasawa, Layered materials with coexisting acidic and basic sites for catalytic one-pot reaction sequences, J. Am. Chem. Soc., 2009, 131(23), 7944–7945 CrossRef CAS PubMed.
- J. Park, J.-R. Li, Y.-P. Chen, J. Yu, A. A. Yakovenko, Z. U. Wang, L.-B. Sun, P. B. Balbuenab and H.-C. Zhou, A versatile metal–organic framework for carbon dioxide capture and cooperative catalysis, Chem. Commun., 2012, 48, 9995–9997 RSC.
- B. Li, D. Ma, Y. Li, Y. Zhang, G. Li, Z. Shi, S. Feng, M. J. Zaworotko and S. Ma, Dual functionalized cages in metal–organic frameworks via stepwise postsynthetic modification, Chem. Mater., 2016, 28, 4781–4786 CrossRef CAS.
- Y. Zhang, Y. Wang, L. Liu, N. Wei, M.-L. Gao, D. Zhao and Z.-B. Han, Robust bifunctional lanthanide cluster based metal–organic frameworks (MOFs) for tandem deacetalization–Knoevenagel reaction, Inorg. Chem., 2018, 57, 2193–2198 CrossRef CAS PubMed.
- J. Qiao, B. Zhang, X. Yu, X. Zou, X. Liu, L. Zhang and Y. Li, A stable Y(III)-based amide-functionalized metal–organic framework for propane/methane separation and Knoevenagel condensation, Inorg. Chem., 2022, 61, 3708–3715 CrossRef CAS PubMed.
- P. Wu, J. Wang, Y. Li, C. He, Z. Xie and C. Duan, Luminescent sensing and catalytic performances of a multifunctional lanthanide–organic framework comprising a triphenylamine moiety, Adv. Funct. Mater., 2011, 21, 2788–2794 CrossRef CAS.
- C. Yao, S. Zhou, X. Kang, Y. Zhao, R. Yan, Y. Zhang and L. Wen, A cationic Zinc–organic framework with Lewis acidic and basic bifunctional sites as an efficient solvent-free catalyst: CO2 fixation and Knoevenagel condensation reaction, Inorg. Chem., 2018, 57(17), 11157–11164 CrossRef CAS PubMed.
- T. Jing, L. Chen, F. Jiang, Y. Yang, K. Zhou, M. Yu, Z. Cao, S. Li and M. Hong, Fabrication of a robust lanthanide metal–organic framework as a multifunctional material for Fe(III) detection, CO2 capture, and utilization, Cryst. Growth Des., 2018, 18, 2956–2963 CrossRef CAS.
- J. Duan, M. Higuchi, R. Krishna, T. Kiyonaga, Y. Tsutsumi, Y. Sato, Y. Kubota, M. Takkata and S. Kitagawa, High CO2/N2/O2/CO separation in a chemically robust porous coordination polymer with low binding energy, Chem. Sci., 2014, 5, 660–666 RSC.
- R. Zhong, X. Yu and R. Zou, A highly thermal stable microporous lanthanide–organic framework for CO2 sorption and separation, Inorg. Chem. Commun., 2015, 61, 17–3176 CrossRef.
- M. Tian, J. Zheng, J. Xue, X. Pan, D. Zhou, Q. Yao, Y. Li, W. Duan, J. Su and X. Huang, A series of microporous and robust Ln-MOFs showing luminescence properties and catalytic performances towards Knoevenagel reactions, Dalton Trans., 2021, 50, 17785–17791 RSC.
- Q. Yao, M. Tian, Y. Wang, Y. Meng, J. Wang, Q. Yao, X. Zhou, H. Yang, H. Wang, Y. Li and J. Zhang, A robust, water-stable, and multifunctional praseodymium–organic framework showing permanent porosity, CO2 adsorption properties, and selective sensing of Fe3+ ion, Chin. J. Struct. Chem., 2020, 39, 1862–1870 Search PubMed.
- Q. Yao, A. B. Gómez, J. Su, V. Pascanu, Y. Yun, H. Zheng, H. Chen, L. Liu, H. N. Abdelhamid, B. Martín-Matute and X. Zou, Series of highly stable isoreticular lanthanide metal–organic frameworks with expanding pore size and tunable luminescent properties, Chem. Mater., 2015, 27, 5332–5339 CrossRef CAS.
- Y. Ye, S. Xiong, X. Wu, L. Zhang, Z. Li, L. Wang, X. Ma, Q.-H. Chen, Z. Zhang and S. Xiang, Microporous metal–organic framework stabilized by balanced multiple host-couteranion hydrogen-bonding interactions for high-density CO2 capture at ambient conditions, Inorg. Chem., 2016, 55, 292–299 CrossRef CAS PubMed.
- A. M. Bohnsack, I. A. Ibarra, P. W. Hatfield, J. W. Yoon, Y. K. Hwang, J.-S. Chang and S. M. Humphrey, High capacity CO2 adsorption in a Mg(II)-based phosphine oxide coordination material, Chem. Commun., 2011, 47, 4899–4901 RSC.
- O. C. Gagné and F. C. Hawthorne, Empirical Lewis acid strengths for 135 cations bonded to oxygen, Acta Crystallogr., 2017, B73, 956–961 Search PubMed.
- P. L. Arnold, M. W. McMullon, J. Rieb and F. E. Kühn, C–H bond activation by f-block complexes, Angew. Chem., Int. Ed., 2015, 54, 82–100 CrossRef CAS PubMed.
- T. Zhang and W. Lin, Metal–organic frameworks for artificial photosynthesis and photocatalysis, Chem. Soc. Rev., 2014, 43, 5982–5993 RSC.
- M. Almáši, V. Zeleňák, M. Opanasenko and I. Císarová, Ce(III) and Lu(III) metal–organic frameworks with Lewis acid metal sites: preparation, sorption properties and catalytic activity in Knoevenagel condensation, Catal. Today, 2015, 243, 184–194 CrossRef.
- G. M. Sheldrick, Crystal structure refinement with SHELXL, Acta Crystallogr., 2015, c71, 3–8 CrossRef PubMed.
- O. V. Dolomanov, L. J. Bourhis, R. J. Gildea, J. A. K. Howard and H. Puschmann, OLEX2: a complete structure solution, refinement and analysis program, J. Appl. Crystallogr., 2009, 42, 339–341 CrossRef CAS.
|
This journal is © The Royal Society of Chemistry 2022 |
Click here to see how this site uses Cookies. View our privacy policy here.