Poly(vinyl acetate-co-crotonic acid) from bio-based crotonic acid: synthesis, characterization and carbon footprint evaluation†
Received
10th February 2023
, Accepted 26th May 2023
First published on 9th June 2023
Abstract
The sludge from wastewater treatment systems (WWTS) contains a high quantity of organic material with great potential for synthesising bio-based chemicals and materials that can replace their fossil-based equivalents. Herein, we have investigated the upcycling of WWTS to the production of bio-based crotonic acid (CA), which is in turn used in replacement of the commercial, fossil-based CA in the copolymerization with vinyl acetate to poly(vinyl acetate-co-crotonic acid). The results demonstrate that a great benefit in terms of GHG emission reduction is obtained when the fossil-based synthesis is replaced with the WWTS-to-CA route, decreasing the carbon footprint of CA from 13.9 to 7.75 kg CO2 per kg CA. The copolymers constituted from fossil-based or bio-based CA have the same composition and thermal properties, independently of the origin and the purity of the starting CA monomer, suggesting their equivalency for the investigated application.
Sustainability spotlight
The treatment of industrial and urban wastewater is a fundamental practice devoted to ensuring public health and environmental protection but generates a huge amount of sludge that must be disposed of. Sludge treatment is a costly operation that includes few management options (i.e., landfilling, incineration, composting, direct usage in agriculture). Novel disposal approaches that can valorise sludge as a feedstock for synthesising renewable chemicals would be valuable in terms of circular economy; in this context, we envisioned the use of the carbon atoms inside sludge for producing a monomer, crotonic acid, polymerizable with vinyl acetate to give a copolymer produced nowadays exclusively from fossil resources. This work aligns with the UN SDG of ensuring sustainable consumption and production patterns.
|
Introduction
The upcycling of waste into added-value products (e.g., bio-based products and bioenergy) is crucial to develop a sustainable and economically feasible bioeconomy. However, the potential use and the actual exploitation of carbon atoms contained in some waste to produce added-value products are often hampered by the chemo-physical characteristics of the waste itself. A paradigmatic example is represented by the sludge originating from the treatment of industrial and urban wastewater (i.e. wastewater treatment sludge, WWTS), whose production derives from a fundamental practice devoted to ensuring public health and environmental protection. The amount of urban and industrial WWTS produced in Europe is about 6 and 4 Mt y−1 on a dry matter basis,1,2 respectively, which corresponds to a huge amount of organic matter potentially available as feedstock for producing bio-based molecules and materials. However, WWTS has a low concentration of soluble organic chemicals, corresponding in terms of chemical oxygen demand (COD) to about 5 g COD per L,3 of which a limited portion is bioavailable for further transformations. The majority of carbon atoms that can be effectively exploited, and therefore the majority of sludge COD, is stored in the solid fraction of WWTS, organized in macromolecules that are recalcitrant to (bio)chemical transformations. Therefore, the solubilization of the COD of WWTS is the rate-limiting step in the production of bio-based chemicals, making the application of pre-treatments fundamental; high-pressure thermal hydrolysis (at 130–180 °C, 6–12 bar) is surely the most mature pre-treatment technology applied at an industrial scale for increasing the soluble COD of WWTS (e.g., Cambi THP, Exelys, Digelis).4 Among the marketable commodities achievable from the conversion of the COD contained in WWTS there are examples of bioenergy carriers (e.g., bio-methane), “drop-in” chemicals such as volatile fatty acids (VFAs) and their esters,5 and “emerging” biopolymers such as polyhydroxyalkanoates (PHA).6 The production of fertilizers and binders from the conversion of the inorganic content of WWTS has been also described in the literature.7,8 Independently from the approach adopted and the feedstock of origin, the value of the bio-based products can be much higher than that of bioenergy, economically pushing the conversion routes of bio-based feedstocks towards the production of the former product rather than the latter one:9 for example, from the direct comparison between PHA and bio-methane, CH4, the prices are >4.5 € per kgPHA and <1 € per kgCH4, respectively.10 In particular, the drop-in synthesis of bio-based products in the short to mid-term can have a much larger impact at the industrial level than the synthesis of completely new biomolecules or biopolymers (i.e., PHA), by-passing some of the critical barriers to commercial development, such as the market acceptance, the rate at which new bio-products may be introduced into the market, the process scalability, and the possibility to use the existing assets and plants for the production.11 On the other hand, the main requirements that drop-in products should have for entering the market are a competitive price, comparable technical performances and a better environmental profile (i.e., lower environmental footprint) than the fossil-based alternatives.12 Here, our interest is oriented to crotonic acid (CA), a drop-in compound that can be obtained both from a fossil-based production route and from the depolymerization of PHA. CA is industrially obtained by oxidation of crotonaldehyde through a process that consists of several stages:13 oil extraction, steam cracking to ethylene and mixture of hydrocarbons, oxidation to acetaldehyde (Wacker process), aldol condensation reaction to produce acetaldol, dehydration to crotonaldehyde, its subsequent oxidation to crotonic acid, and its final purification through distillation and crystallization (Fig. 1). Bio-based CA can be produced from polyhydroxybutyrate (PHB), a homopolymer of the PHA family; the thermal degradation of PHB occurs through β-elimination reactions that randomly break the chains and give the trans isomer of CA as the major product (Fig. 1). The by-products of such depolymerization include the cis isomer of CA (isocrotonic acid), 3-butenoic acid (vinylacetic acid), alkenyl-terminated dimers and trimers, and crotonamide (in the case of PHB inclusions inside bacterial cells); the selectivity towards trans-CA is drastically improved if alkaline catalysts like Mg(OH)2, MgO or CaO are applied during the thermal treatment.14–18CA finds application in coatings, paints, textiles, binders, adhesives, flocculants, ceramics, and agrochemicals, with a global production of 50–70 kt y−1, a global market of about 600 M€, and a selling price of 7–10 € per kg.19 The main industrial use of CA is as a building block in the synthesis of poly(vinyl acetate-co-crotonic acid), the copolymer with vinyl acetate (VA),13 since it is quite suitable to polymerization in analogy to other α,β-unsaturated compounds (e.g. acrylic acid) (Fig. 1). Poly(vinyl acetate-co-crotonic acid) (VA–CA), with trade name Mowilith, Vinnapas, Vinaflex, and Vinac, has a CA content of 1–5% mol and finds application as an ingredient for the preparation of low-profile additives (LPAs) for the automotive market.13 Currently, both vinyl acetate and crotonic acid used for synthesising poly(vinyl acetate-co-crotonic acid) have a 100% fossil origin, even if it is worth mentioning that Wacker Chemie, one of the suppliers of poly(vinyl acetate-co-crotonic acid), introduced bio-based acetic acid from the wood-processing industry in some of its VA-based products.20 In our previous work, we have already demonstrated the route “WWTS-to-PHB” by using a hybrid thermochemical–biological approach that combines (i) the hydrothermal carbonization (HTC) of WWTS, (ii) the anaerobic fermentation of the HTC-treated WWTS into VFAs, and lastly (iii) the aerobic biological conversion of VFAs into PHB.21 Moreover, we have developed a “thermolytic distillation” process capable of depolymerizing PHB or PHB inclusions into CA at high yields and purity.22 The thermolytic distillation process that we developed does not require catalysts, nor harsh conditions (210 °C and 150 mbar), and it consumes 10–20% less energy than previously reported depolymerization methods.23 Herein we aim at demonstrating that bio-based CA produced by the route “WWTS-to-CA” can replace fossil-based CA, giving the same technical performances in the copolymerization with VA to synthesise poly(vinyl acetate-co-crotonic acid) while improving the carbon footprint of the final copolymer. To this purpose, we polymerized VA with CA samples produced from (i) PHB inclusions inside bacteria cultivated by using WWTS as feedstock21,22 and (ii) pure PHB,22 providing the first example of poly(vinyl acetate-co-crotonic acid) in which CA is synthesised from renewable resources.
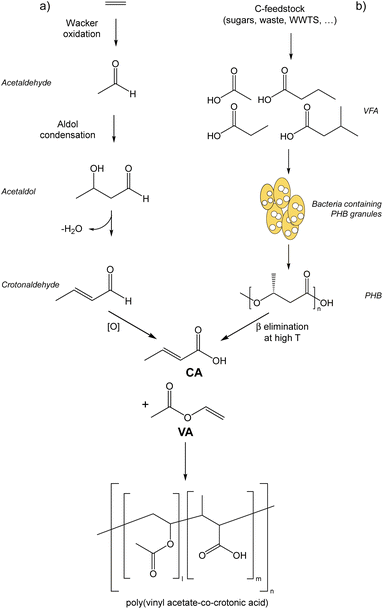 |
| Fig. 1 Synthetic pathways for producing CA from (a) fossil resources, and (b) renewable feedstock, and the following co-polymerization with VA to give poly(vinyl acetate-co-crotonic acid). | |
Results and discussion
Synthesis of poly(vinyl acetate-co-crotonic acid)
A literature survey surprisingly highlights the lack of an optimized procedure for the preparation of poly(vinyl acetate-co-crotonic acid).24 However, when VA is used as a comonomer, emulsion polymerization in water in the presence of an initiator and an emulsifier agent is mainly adopted.25 We then planned a synthesis under batch conditions by adapting related polymerization procedures previously reported.26 Thus, VA (2 mL, ρ = 0.93 g mL−1, 1.86 g) was polymerized with the chosen CA sample (28 mg, 1.5 wt%, see Fig. S1 in ESI†) in the presence of benzoyl peroxide as initiator and polyvinyl alcohol as an emulsifier (see Table S1 in ESI†). These unoptimized experiments were intended to find those parameters leading to the highest mass balance by tuning the loading of the initiator (0.65–2.5 wt%), and of the emulsifier (0.01–0.05 wt%), along with the reaction temperature (60–100 °C) and the reaction time (3–16 h). Significant amounts of polymer have been formed only when heating the reaction mixture at least at 100 °C for 6 h (see Table S1 in ESI†). The best polymerization conditions were then used for the synthesis of three poly(vinyl acetate-co-crotonic acid) samples containing bio-based CA (Fig. 2). The samples included a copolymer in which CA came from the thermolytic distillation of pure commercial PHB (CAPHB, purity of 98%)22 and two copolymers in which CA derived from the thermolytic distillation of bacteria containing 30 and 60% of PHB and fed with hydrothermally treated and anaerobically digested WWTS (CA30 and CA60, purity of 92 and 96%, respectively; see ref. 21 for details about WWTS pre-treatment and bacterial cultivation).22
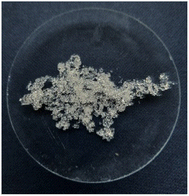 |
| Fig. 2 Poly(vinyl acetate-co-crotonic acid) beads prepared with bio-based crotonic acid (CA60). | |
These samples were analysed and compared with the copolymer produced by adopting the same copolymerization procedure starting from fossil-based CA (hereafter named CAc) and VA. The features of the polymers formed were compared with those of Vinaflex, one of the co-polymers on the market, for the sake of comparison, despite the latter sample was expected to show better performance due to the optimized conditions used for its preparation at the industrial scale.
Characterization of poly(vinyl acetate-co-crotonic acid)
All the copolymers were characterized first by gel permeation chromatography (GPC) to assess the molecular weight and polydispersity achieved after purification via subsequent precipitation in cyclohexane. The mean molecular weight
values found are independent of the type of CA used (Table 1 and Fig. S3 in ESI†), even if markedly lower than the value of the commercial sample. All the 1H-NMR spectra (see Fig. S4 in ESI†) show broad signals as expected upon polymeric backbone formation; furthermore, all the copolymers spectra show a pattern comparable to the literature data27 and the commercial poly(vinyl acetate-co-crotonic acid), suggesting that the applied polymerization route gave polymers similar to the commercial reference in terms of comonomers inclusion. The elemental composition of the synthesised copolymers and the commercial one is not statistically different, and no traces of N, imputable to crotonamide contamination, were found in the sample containing CA30.22 The molar composition of the copolymers determined by comparing the integral values of VA CH and CA CH3, suggests that all synthesised copolymers are richer in CA than the commercial one (ca. 2.5 mol% vs. 0.7 mol%) (Table 1). This point might have to do with the lower molecular weight of the copolymers achieved by applying an unoptimized procedure at a lab scale but helped us to highlight the effect of CA characteristics on its conversion. The origin and the purity of the starting CA monomer did not affect the final copolymer composition when polymerization conditions are kept unchanged. Polyvinyl acetate (PVA) homopolymer is known to exhibit a two-stage decomposition,28 the first stage of which, between 260 and 380 °C, produces a large amount of acetic acid. Deacetylation can occur intramolecularly with the intermediate formation of a six-member cyclic product paving the way to subsequent aromatization.29,30 The thermogravimetric analysis (TGA) of the commercial poly(vinyl acetate-co-crotonic acid) (Table 1 and Fig. S5 in ESI†) shows a behaviour reminiscent of the prevailing VA component homopolymer, with a two-step weight loss and a carbonaceous residue at the end of the run corresponding to 5% of the initial weight of the sample. The same weight loss is observed for all the here synthesised copolymers, and it is mainly due to the inert atmosphere applied that fosters unsaturation formation and in turn aromatization in the absence of oxygen. All the synthesised copolymers show a similar thermal pattern (Table 1), with only the one synthesised from CAc displaying a slightly lower Tonset for the degradation, which might stem from the higher CA content in the backbone. Differential scanning calorimetry (DSC) analysis shows for all the obtained copolymers only a stepwise transition that can be attributed to the glass transition (Tg) around 46–47 °C (Fig. 3). As expected for atactic polymers as the ones presently investigated, the lack of endothermic peaks rules out any crystallization phenomena. The commercial reference shows a slightly lower Tg possibly due once again to the different composition. Anyway, the net presence of a single transition corroborates the hypothesis of CA incorporation within the main polymeric backbone.
Table 1 Physical characteristics of poly(vinyl acetate-co-crotonic acid) obtained through copolymerization of vinyl acetate (VA) and various sources of CA (
: mean molecular weight; PDI: polydispersity index; Td: degradation temperature determined as the onset of the first weight loss as measured by TGA; Tg: glass transition temperature)
Poly(vinyl acetate-co-crotonic acid) |
 (kDa) |
PDI |
Molar ratio (mol%) |
T
d (°C) |
T
g (°C) |
Elemental composition (%) |
CA
|
VA
|
N |
C |
H |
Comm. |
184 |
1.9 |
0.7 |
99.3 |
316 |
43 |
— |
52.5 ± 0.2 |
6.7 ± 0.05 |
VA–CAc |
25 |
2.0 |
2.6 |
97.4 |
312 |
47 |
— |
54.1 ± 1.4 |
6.8 ± 0.1 |
VA–CAPHB |
27 |
2.2 |
2.3 |
97.7 |
313 |
46 |
— |
52.8 ± 1.4 |
6.7 ± 0.6 |
VA–CA30 |
33 |
2.1 |
2.3 |
97.7 |
313 |
46 |
— |
53.5 ± 1.7 |
6.8 ± 0.4 |
VA–CA60 |
27 |
2.4 |
2.6 |
97.4 |
313 |
47 |
— |
52.2 ± 0.9 |
6.6 ± 0.07 |
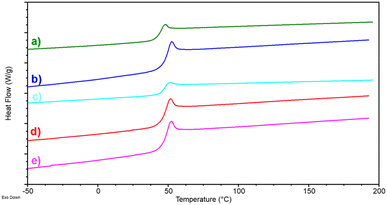 |
| Fig. 3 DSC traces of the second heating scan for (a) the commercial polymer (green line), and for the four synthesised copolymers: (b) VA–CAc (blue line), (c) VA–CAPHB (light blue line), (d) VA–CA30 (red line), (e) VA–CA60 (pink line). | |
Carbon footprint estimation
A preliminary carbon footprint evaluation was carried out to estimate the potential contribution to greenhouse gas (GHG) emissions savings of the bio-based production of CA compared to the fossil-based CA production currently in place. For bio-based CA, the use of WWTS from the food industry is considered as feedstock (30 g L−1 of COD and a dry matter of 4 wt%) to the upcycling process consisting of the following stages (see Table S2 in ESI†):
(i) Hydrothermal carbonization (HTC) pre-treatment of WWTS at 200 °C (18 bar of auto-generated pressure) to give a hydrochar and an aqueous phase enriched in fermentable organic compounds like sugars and short-chain carboxylic acids;
(ii) Anaerobic digestion of the entire pre-treated WWTS under thermophilic conditions (55 °C) to give VFAs;
(iii) Solid/liquid separation of the mixture through a filter-press to get a VFAs enriched solution and a “cake” with a high dry matter content (about 40 wt%) composed of digestate and hydrochar;
(iv) Aerobic fermentation of the VFA stream to produce PHB as intracellular granules (45% on bacterial biomass basis, as the average between 30 and 60%);
(v) Drying of the microbial biomass enriched in PHB, followed by its thermolytic distillation at 210 °C and 150 mbar to give bio-based CA.
No additional purification step was included in the modelling since the characterization of the copolymers produced from bio-based CA samples here reported highlighted that neither the origin nor the purity of CA affected the final copolymer.
All the assumptions and the estimation of the energy consumption of the various steps have been derived from the running of a semi-pilot scale plant realized within the B-PLAS DEMO project (see Table S2 in ESI† for details).31
The life cycle assessment (LCA) analysis demonstrates a GHG emission reduction from 13.9 kg CO2 eq. per kg CA to 7.75 kg CO2 eq. per kg CA in favour of the replacement of fossil sources with the WWTS upcycling route here proposed (Fig. 4 and 5). The main contribution to such carbon emission reduction is due to much lower steam inputs to the depolymerization of PHB through thermolytic distillation (210 °C, 150 mbar) than those required for fossil-based CA (Fig. 4). For bio-based CA, the most contributing stage to carbon emissions is HTC due to intensive thermal energy requirements. In contrast, anaerobic fermentation is given a credit for the recovery of energy during the combustion in a combined heat and power (CHP) plant of the biogas produced.
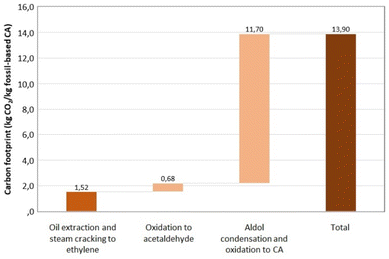 |
| Fig. 4 Carbon footprint estimate for 1 kg of CA from fossil sources, absolute value and breakdown by main life cycle stage. Own calculation based on IDEA and Ecoinvent databases.32,33 | |
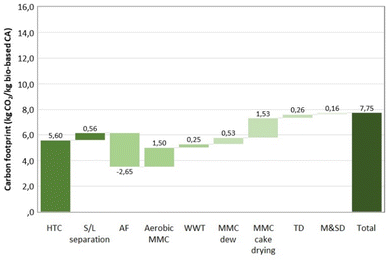 |
| Fig. 5 Carbon footprint estimate for 1 kg of bio-based CA, absolute value and breakdown by main life cycle stage. Own calculation based on chemical plant modelling at the semi-pilot scale.31 HTC – hydrothermal carbonization; S/L – solid/liquid separation; AF – anaerobic fermentation; MMC – mixed microbial cultures; WWT – wastewater treatment; TD – thermolytic distillation; M & SD – monitoring and safety design. | |
Despite preliminary, these results highlight a significant potential for carbon savings achievable by a bio-based production process of CA, which will be ultimately embedded into the environmental profile of its derived copolymer poly(vinyl acetate-co-crotonic acid). It is worth noting that the WWTS-to-CA process provides the supplemental function of dewatering the sludge input thanks to the application of HTC: during HTC, 40–60% of WWTS is converted into a carbon-densified product (hydrochar) containing most of the suspended solids in the sludge coming out of the HTC reactor. For this reason, it can be easily filtered at high pressure with a filter press, getting an effective dehydration result impossible to get without HTC and ultimately reducing the amount of waste to be disposed of (i.e. the dry matter increases from 4 wt% in the sludge in input to about 40–60 wt% in the sludge in output). To include this further benefit into our preliminary assessment, we have expanded the system boundaries to include and compare the main end-of-life (EoL) scenarios for managing WWTS and normalized the results to a reference flow of 1 tonne of dry sludge (tdry WWTS). Based on a review of 35 LCA studies,34 the carbon footprint of the most common WWTS management strategies including direct land application (hereafter, Scenario 1), incineration (Scenario 2), and composting (Scenario 3) has been quantified at 1.3 ± 1.7, 0.42 ± 0.52, and 0.028 ± 0.12 t CO2 per tdry WWTS, respectively. Literature studies point out how local conditions such as the determination of physical–chemical characteristics of sludge inputs, quantification of fugitive emissions from WWTS management options, and modelling of disposal routes may significantly influence the overall environmental performance of sludge use on land or incineration. Further discrepancies may also result from assumptions of system expansion to account for the avoided environmental impacts from material and energy recovery.
Examples include crediting for the use of sludge as a source of N, P and K to offset the consumption of nutrients from fossil origin, or for energy recovery from incineration to offset the generation of electrical and thermal energy from national energy mixes. Ultimately, these technical assumptions explain the great variability of the results. Scenarios 1–3 usually require sludge thickening (in which the dry matter increases from 2 to 4 wt%) and a subsequent stage of solid/liquid separation to reach 25% of dry matter (and a polyelectrolyte consumption of ∼8 kg per tdry WWTS), before composting the dehydrated sludge or disposing of it in a direct land application or incineration plant. The carbon footprint related to dewatering (about 17 kg CO2 eq. per tdry WWTS)35 is avoidable in the WWTS-to-CA process here described. Under the hypothesis that the bio-based CA production process is enforced after thickening and anaerobic digestion stages, a credit of about 190 kg CO2 eq. per tdry WWTS can be subtracted from the carbon footprint of the different EoL scenarios for WWTS, leading to an average reduction of 14% for direct use on land, 45% for incineration and 679% for composting. Furthermore, a considerable reduction in the volume of WWTS to be treated, if compared to that produced from the solid/liquid separation step in the technology currently in place, would enable a more efficient waste transport (i.e., a higher amount of loaded sludge per vehicle) over the same distance covered, ultimately reducing the overall carbon footprint associated with EoL treatment of the sludge. The preliminary environmental impact assessment of the bio-based CA demonstrates the substantial potential for climate mitigation achievable through the WWTS-to-CA valorisation. These estimates will be used as a quantitative basis for further refinement of the avoided impacts related to (i) the production of CA from fossil sources, (ii) the dewatering of digestate, and (iii) the transport of a higher quantity of treated sludge to the final disposal site.
Conclusion
The main renewable resources available on the market for synthesising bio-based building blocks belong to the so-called “first-generation biomass”, like sugars/polysaccharides and vegetable oils. The sugar platform, in particular, is the direct precursor for almost all C2–C4 “drop-in” bio-based chemicals, even if their exploitation for such purpose is a strategy destined to be disincentivized in the next future.36 It is imperative to design bio-based products starting from less problematic renewable feedstocks than biomass characterized by a high indirect land use change (ILUC)-risk, like waste, and develop synthetic approaches that can recycle waste C atoms into new carbon-based products. Herein we have demonstrated that it is possible to mine C atoms from WWTS and produce a C4 carboxylic acid (i.e., CA), that can enter the market with the same technical performance as fossil-based CA but almost halving the related GHG emissions. Poly(vinyl acetate-co-crotonic acid) obtained from various bio-based CA sources had a comparable thermal behaviour to the copolymer containing fossil-based CA, independently of the origin and the purity; moreover, the copolymer that contains bio-based CA improves its overall environmental profile thanks to a significant carbon saving achievable by producing one of the two monomers starting from WWTS through the integrated (thermo)chemical–biological strategy here applied. It is apparent, however, that the environmental benefit of the process is related to the quantitative conversion of the monomers. Work in this direction will be reported in due course.
Experimental
Chemicals and materials
All reagents, solvents and chemicals used in this work were of analytical grade and purchased from various commercial suppliers. Commercial PHB with a mean molecular weight of 0.8 MDa and a polydispersity index of 5.9 was bought from Biomer (Germany). Commercial CA (i.e. fossil-based, CAc) was purchased from Sigma-Aldrich and had a purity of 98%. Commercial poly(vinyl acetate-co-crotonic acid) (Vinaflex) was kindly provided by Vinavil (Italy). Freeze-dried microbial biomass containing 30 and 60% of PHB was produced according to the literature;22 commercial PHB and PHB-enriched biomass were subjected to thermolytic distillation (210 °C, 150 mbar) to produce three samples of CA: CAPHB, CA60 and CA30. The purity and the yield of all the CA achieved were in line with what we have previously reported.19
Synthesis of poly(vinyl acetate-co-crotonic acid)
The poly(vinyl acetate-co-crotonic acid) samples were prepared by heating vinyl acetate (VA; 2 mL, ρ = 0.93 g mL−1, 1.86 g; 0.01 mol) and the chosen sample of crotonic acid (CA; 28 mg, 1.5 wt%) in 4 mL of water, in the presence of 24 mg benzoyl peroxide (1.3 wt%) and 10 mg (0.05 wt%) of polyvinyl alcohol at 100 °C for 6 h (see the experimental setup gathered in Fig. S2 in ESI†). After cooling down the reaction mixture at room temperature, while maintaining a constant stirring, the polymer was isolated as beads through filtration under vacuum and dried at room temperature (see Fig. 2). Polymer samples were dissolved in chloroform and lastly precipitated in cyclohexane before their characterization.
Characterization of poly(vinyl acetate-co-crotonic acid)
Elemental analysis.
The elemental composition of commercial poly(vinyl acetate-co-crotonic acid) and the copolymers here synthesised was determined using an elemental analyzer (Thermo Scientific, Flash 2000, Organic Elemental Analyzer) through the flash combustion technique.
Molecular weight determination.
The average molecular weight and polydispersity of the poly(vinyl acetate-co-crotonic acid) samples were determined by size exclusion chromatography (SEC) in CHCl3 using an HPLC Lab Flow 2000 apparatus working with a 1 mL min−1 flow, equipped with an injector Rheodyne 7725i, a Phenomenex Phenogel 5u MXM column and a RI detector Knauer RI K-2301. Each sample was filtered with a 0.45 μm porosity Teflon filter and the sample injection volume was set to 20 μL. Calibration curves were obtained using several monodisperse polystyrene standards in the range of 13.3–214 kDa.
Thermal analysis.
Thermogravimetric (TGA) measurements were carried out using a Netzsch Vacuum-tight Thermo Micro Balance system TG 209 F1. The analyses were performed at 10 °C min−1 from room temperature to 600 °C under a nitrogen flow. Degradation temperature (Td) is defined as the extrapolation of the onset temperature of the first significant degradation process occurring. Thermal transitions were measured using a differential scanning calorimeter (DSC Q2000; TA Instruments), equipped with a refrigerated cooling system (RCS). Samples, under nitrogen flow, were subjected to a first heating scan at 10 °C min−1, from −90 to 200 °C, to erase polymer thermal history, then cooled at −10 °C min−1 to −90 °C and subsequently subjected to a second heating scan to investigate thermal properties.
NMR analysis.
1H NMR spectra were recorded on a 400 MHz spectrometer and acquired using CDCl3 as the solvent. Chemical shifts are given in ppm relative to tetramethylsilane (TMS).
Carbon footprint estimation.
A preliminary life cycle assessment (LCA) comparison between the carbon footprint of bio-based CA and fossil-based CA has been carried out. To this aim, LCA models were created according to the ISO Standards 14040-14044.37,38
“Cradle-to-gate” system boundaries were set to cover the main life cycle phases from raw material extraction to the production of 1 kg CA, which is set as the functional unit. Life cycle inventories were developed for fossil-based and bio-based CA, respectively. More in detail, fossil-based CA has been modelled using data gathered from the existing literature and databases for LCA. Specifically, Ecoinvent 3.3 (ref. 33) provided the dataset of material and energy inputs and outputs by process, from oil extraction to acetaldehyde production. Further transformation of acetaldehyde to purified CA has been modelled using, instead, the process inventory reported in the IDEA database.32
For bio-based CA, the biological–thermochemical process has been developed based on the modelling of the system parameters, including the main material and energy inputs and outputs, at the semi-pilot scale (see Table S2 in ESI†). While materials (e.g., reagents) required for the synthesis of CA have been assumed to be supplied from the global market, Italy-specific adjustments have been applied to background processes for electrical energy generation to adjust LCA unit processes to the spatial boundary of the process under scrutiny. No environmental impacts have been assumed for the generation of WWTS following the “zero burdens” approach. To enable comparison with the results for Scenario 1–3, the carbon footprint of the WWTS upcycling route (CFWWTS) has been corrected for the avoided dewatering step as follows:
where CF
bCA is the carbon footprint of bio-based
CA produced from WWTS (
i.e., 7.75 kg CO
2 eq. per kg
CA), CF
fCA is the carbon footprint of the fossil-based
CA (13.9 kg CO
2 eq. per kg
CA),
mCA is the amount of
CA produced by treating 1000 t WWTS with a DM of 4 wt% (1200 kg), CF
dew is the carbon footprint of the solid/liquid separation to reach 25% of dry matter (17.0 kg CO
2 eq. per t
dry matter) (see Table S2 in ESI
† for the calculations).
The SimaPro software was used for system modelling, while the IPCC GWP100a assessment method was applied for the carbon footprint evaluation (ISO 14067:2018).39
Author contributions
T. B., A. J., L. M., A. P., D. R., L. C., C. T.: methodology, data curation, investigation (A. P.: design and development of thermolytic distillation; A. J. and D. R.: synthesis of poly(vinyl acetate-co-crotonic acid); T. B. and L. M.: characterization of poly(vinyl acetate-co-crotonic acid); L. C. and C. T.: carbon footprint estimation). D. R., L. M., L. C., C. S.: conceptualization, writing-original draft preparation, writing-reviewing and editing, supervision. P. G., M. F., I. V.: writing-reviewing and editing. D. R., C. T., C. S., M. F., P. G., I. V., L. C.: funding acquisition.
Conflicts of interest
There are no conflicts to declare.
Acknowledgements
The authors gratefully acknowledge financial support from the European Union's Life Programme under grant agreement no. 101074164 (CROSS-LIFE – CROtonic Acid from Sewage Sludge). Views and opinions expressed are however those of the author(s) only and do not necessarily reflect those of the European Union or CINEA. Neither the European Union nor the granting authority can be held responsible for them. This study was partially carried out within the MICS (Made in Italy – Circular and Sustainable) Extended Partnership and received funding from the European Union Next-GenerationEU (PIANO NAZIONALE DI RIPRESA E RESILIENZA (PNRR) – MISSIONE 4 COMPONENTE 2, INVESTIMENTO 1.3 – D.D. 1551.11-10-2022, PE00000004). This manuscript reflects only the authors' views and opinions, neither the European Union nor the European Commission can be considered responsible for them. The PhD project of A. J. was supported by the “PON Ricerca e Innovazione” program (action IV.5; D.M. 1061-2021). The authors acknowledge Vinavil S.p.A. (Italy) for the commercial sample of poly(vinyl acetate-co-crotonic acid) and Diemme filtration (Aqseptence group, Italy) for the filter press used in the project B-PLAS DEMO.
Notes and references
- A. Kelessidis and A. S. Stasinakis, Waste Manage., 2012, 32, 1186–1195 CrossRef CAS PubMed.
- O. Vigiak, B. Grizzetti, A. Udias-Moinelo, M. Zanni, C. Dorati, F. Bouraoui and A. Pistocchi, Sci. Total Environ., 2019, 666, 1089–1105 CrossRef CAS PubMed.
- A. Donoso-Bravo, S. Pérez-Elvira, E. Aymerich and F. Fdz-Polanco, Bioresour. Technol., 2011, 102, 660–666 CrossRef CAS PubMed.
- K. Hii, S. Baroutian, R. Parthasarathy, D. J. Gapes and N. Eshtiaghi, Bioresour. Technol., 2014, 155, 289–299 CrossRef CAS PubMed.
- S. Longo, E. Katsou, S. Malamis, N. Frison, D. Renzi and F. Fatone, Bioresour. Technol., 2015, 175, 436–444 CrossRef CAS PubMed.
- G. Mannina, D. Presti, G. Montiel-Jarillo, J. Carrera and M. E. Suárez-Ojeda, Bioresour. Technol., 2020, 297, 122478 CrossRef CAS PubMed.
- M. Franz, Waste Manage., 2008, 28, 1809–1818 CrossRef CAS PubMed.
- L. Świerczek, B. M. Cieślik and P. Konieczka, J. Cleaner Prod., 2021, 287, 125054 CrossRef.
- A. Alloul, R. Ganigué, M. Spiller, F. Meerburg, C. Cagnetta, K. Rabaey and S. E. Vlaeminck, Environ. Sci. Technol., 2018, 52, 6729–6742 CrossRef CAS PubMed.
-
(a) A. Werker, S. Bengtsson, L. Korving, M. Hjort, S. Anterrieu, T. Alexandersson and F. Morgan-Sagastume, Water Sci. Technol., 2018, 78, 2256–2269 CrossRef CAS PubMed;
(b)
https://ec.europa.eu/eurostat/data/database
.
-
T. Tabanelli and F. Cavani, in Biomass Valorization, ed. D. Ravelli and C. Samorì, Wiley-VCH Verlag GmbH & Co. KGaA, Weinheim, Germany, 2021 Search PubMed.
- R. Mori, RSC Sustainability, 2023, 1, 179–212 RSC.
-
J. Blumenstein, J. Albert, R. P. Schulz and C. Kohlpaintner, in Ullmann's Encyclopedia of Industrial Chemistry, Wiley-VCH Verlag GmbH & Co. KGaA, Weinheim, Germany, 2015, pp. 1–20 Search PubMed.
- H. Ariffin, H. Nishida, Y. Shirai and M. A. Hassan, Polym. Degrad. Stab., 2010, 95, 1375–1381 CrossRef CAS.
-
H. Nishida, H. Ariffin, Y. Shirai and M. Hassan, in Biopolymers, ed. M. Elnashar, IntechOpen, 2010, pp. 369–386 Search PubMed.
- H. Ariffin, H. Nishida, M. A. Hassan and Y. Shirai, Biotechnol. J., 2010, 5, 484–492 CrossRef CAS PubMed.
- J. C. A. Flanagan, J. Myung, C. S. Criddle and R. M. Waymouth, ChemistrySelect, 2016, 1, 2327–2331 CrossRef CAS.
- F. D. Kopinke, M. Remmler and K. Mackenzie, Polym. Degrad. Stab., 1996, 52, 25–38 CrossRef CAS.
-
https://www.researchandmarkets.com
.
-
https://www.wacker.com/cms/enus/products/brands/vinnapas/vinnapas.html
.
- C. Samorì, A. Kiwan, C. Torri, R. Conti, P. Galletti and E. Tagliavini, ACS Sustainable Chem. Eng., 2019, 7, 10266–10273 CrossRef.
- A. Parodi, A. Jorea, M. Fagnoni, D. Ravelli, C. Samorì, C. Torri and P. Galletti, Green Chem., 2021, 23, 3420–3427 RSC.
- C. Samorì, G. A. Martinez, L. Bertin, G. Pagliano, A. Parodi, C. Torri and P. Galletti, Resour., Conserv. Recycl., 2022, 178, 106082 CrossRef.
-
(a) D. Donescu and L. Fusulan, J. Dispersion Sci. Technol., 1996, 17, 631–644 CrossRef CAS;
(b)
W. O Hermann, H. Wolfram and W. Heinz, US Pat., 2855374, 1958 Search PubMed.
-
(a) D. Donescu and L. Fusulan, Acta Polym., 1991, 41, 526–530 CrossRef;
(b) G. S. Magallanes Gonzalez, V. L. Dimonie, E. D. Sudol, H. J. Yue, A. Klein and M. S. El-Aasser, J. Polym. Sci., Part A: Polym. Chem., 1996, 34, 849–862 CrossRef CAS;
(c) H. Egret, V. L. Dimonie, E. D. Sudol, A. Klein and M. S. El-Aasser, J. Appl. Polym. Sci., 2001, 82, 1739–1747 CrossRef CAS.
-
(a)
D. I. Jon and J. C. Hornby, US Pat., 5225474, 1993 Search PubMed;
(b) D. Guan, J. Li, G. Sun and X. Zhai, IOP Conf. Ser.: Mater. Sci. Eng., 2019, 473, 0120126 CrossRef.
- S. Amiya and M. Uetsuki, Macromolecules, 1982, 15, 166–170 CrossRef CAS.
-
I. C. McNeill, in Comprehensive Polymer Science and Supplements, ed G. Eastmond, A. Ledwith, S. Rosso and P. Sigwalt, Pergamon Press, Oxford, 1989, pp. 451–500 Search PubMed.
- N. Grassie, Trans. Faraday Soc., 1952, 48, 379–387 RSC.
- N. Grassie, Trans. Faraday Soc., 1953, 49, 835–842 RSC.
-
B-PLAS DEMO (2018–2021), Funded by EIT-Climate KIC, https://site.unibo.it/b-plas/en Search PubMed.
-
Research Laboratory for IDEA, Research Institute of Science for Safety and Sustainability and National Institute of Advanced Industrial Science and Technology (AIST, Sustainable Management Promotion Organization (SuMPO)), LCI Database IDEA Version 2, https://www.idea-lca.jp/, accessed on January 2023 Search PubMed.
- G. Wernet, C. Bauer, B. Steubing, J. Reinhard, E. Moreno-Ruiz and B. Weidema, Int. J. Life Cycle Assess., 2016, 21, 1218–1230 CrossRef.
- H. Yoshida, T. H. Christensen and C. Scheutz, Waste Manage. Res., 2013, 31, 1083–1101 CrossRef PubMed.
- F. Mayer, R. Bhandari and S. A. Gath, J. Environ. Manage., 2021, 290, 112557 CrossRef CAS PubMed.
-
Communication from the Commission to the European Parliament, The Council, the European Economic and Social Committee and The Committee of the Regions, A Sustainable Bioeconomy for Europe: Strengthening the Connection between Economy, Society and the Environment, 2018, https://eur-lex.europa.eu/legal-content/ Search PubMed.
-
ISO 14040:2006/A1:2020; Environmental Management—Life Cycle Assessment—Principles and Framework, ISO, Geneva, Switzerland, 2020 Search PubMed.
-
ISO 14044:2006/A2:2020; Environmental Management, Life Cycle Assessment, Requirements and Guidelines, ISO, Geneva, Switzerland, 2020 Search PubMed.
-
ISO 14067:2018; Greenhouse Gases—Carbon Footprint of Products—Requirements and Guidelines for Quantification, ISO, Geneva, Switzerland, 2020 Search PubMed.
Footnote |
† Electronic supplementary information (ESI) available: Copolymerization mass balance, 1H-NMR spectra, TGA and GPC curves, assumptions for the calculation of the carbon footprint. See DOI: https://doi.org/10.1039/d3su00052d |
|
This journal is © The Royal Society of Chemistry 2023 |
Click here to see how this site uses Cookies. View our privacy policy here.