DOI:
10.1039/D3MR00025G
(Communication)
RSC Mechanochem., 2024,
1, 38-42
Synthesis of α-ketothioamides with elemental sulfur under solvent-free conditions in a mixer mill†
Received
28th November 2023
, Accepted 5th January 2024
First published on 29th January 2024
Abstract
A mechanochemical base-mediated synthesis of α-ketothioamide from readily available acetophenone derivatives is developed. The reaction is metal-free, solventless, and proceeds in a short reaction time. Importantly, the products differ from those formed under standard solution-based protocols.
Introduction
Organosulfur compounds are structurally diverse and widely used as pharmaceuticals1 and crop protecting agents.2 Among them, thioamides and α-ketothioamides have attracted particular attention as interesting building blocks for a large variety of sulfur-containing compounds and natural products.3 A representative direct application was reported by Frédérick and co-workers, who found inhibitory activities of α-ketothioamide derivatives on phosphoglycerate dehydrogenase (PHGDH) with implications for cancer cell proliferation.4
Three recent methods for accessing α-ketothioamides are shown in Scheme 1. The first (a) is an iron-catalysed oxidative Willgerodt–Kindler-type reaction,5–7 which is of interest because it involves a combination of simple starting materials such as methyl ketones, elemental sulfur,8 and dioxygen. However, for achieving satisfying results, significant amounts of the metal catalyst, an elevated temperature, and an extended reaction time are required limiting the synthetic attractiveness of this process. An advanced version of this method is solvent-free, but relies on sonication at 80 °C for 5 h and the use of an ionic liquid (IL)-modified iron nanoparticle catalyst (Fe3O4@IL-ZnCl2), which is time-consuming to prepare following a multistep procedure.9 In the second approach towards α-ketothioamides (b), sulfoxonium ylides are used as starting material.10 The method is of interest because it neither needs a metal catalyst, nor a base or additive. However, the ylides are commonly prepared from the corresponding acyl chlorides and THF is used as organic solvent, which both can be critical for large-scale applications. The third recently published method (c) makes use of a pre-prepared starting material as well, and also in this case, an organic solvent and high temperature are essential.11 Other methods follow analogous strategies, mostly by activating the methyl group of an acetophenone moiety or by starting from preformed α-functional ketones.3c,8a,12
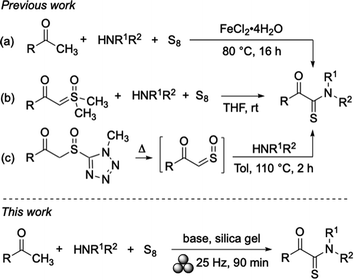 |
| Scheme 1 Recent methods for the preparation of α-ketothioamides. | |
Over the last two decades, mechanochemical techniques have gained increasing attention in the field of organic synthesis, and in numerous studies it has been demonstrated that their applications can facilitate reactions, alter product compositions, lead to shorter reaction times, allow lower reaction temperatures, and improve sustainability metrics compared to solution-based protocols.13 Thus, mechanochemistry is in accordance with the 12 principles of green chemistry, and in 2019 IUPAC selected it as one of the 10 world-changing innovations.14 Herein, we report a simple mechanochemical approach towards α-ketothioamides. It is metal- and solvent-free and allows to convert readily available acetophenone derivatives into the target compounds in a single step after a short reaction time. Noteworthy, the products deviate from the ones observed in solution using the same starting materials. Compared to the existing technology, the protocol is simple, the experiments are easy to perform, and neither high temperature nor other harsh conditions are required. Finally, to the best of our knowledge, this is only the second time that elemental sulfur was used in a mechanochemically activated organic synthesis.15–17 Further studies focused on the use of Lawesson's reagent as a sulfur surrogate in the synthesis of thioamides and thiolactams in the ball mill.18
Results and discussion
Initially, we intended to perform a standard Willgeroth–Kindler (WK) reaction6 under mechanochemical conditions. Thus, starting from acetophenone (1a), morpholine (2a), and elemental sulfur, we expected to obtain thioamide 4aa as major product.19 However, when these components were ground in a 10 mL stainless-steel jar with one ball (10 mm ø) of the same material for 60 min at 25 Hz in a mixer mill, 4aa remained undetected, and instead, α-ketothioamide 3aa was formed, albeit in only a small amount (5% of isolated product, Table 1 entry 1). The addition of silica gel (230–400 mesh) as a grinding auxiliary led to a slight increase in the product yield (10%, Table 1, entry 2). The presence of other acidic,20 basic or metallic additives had no significant effect, and the yields of 3aa remained low (Table 1, entries 3–7). Substituting elemental sulfur by NaSH did also not lead to an improved product formation (Table 1, entry 8). With the intention to activate the ketonic methyl group by iodination, elemental iodine and NIS were added,21 but neither of both compounds had a positive effect on the formation of 3aa (Table 1, entries 9 and 10). Substitution of SiO2 by NaCl as grinding auxiliary showed no improvement and multiple spots on the TLC plate were observed (Table 1, entry 11). The first significant improvement in the formation of 3aa was observed, when NaOH was added to the reaction mixture (Table 1, entry 12). At the same time, the reaction time was extended from 60 min to 90 min, and the amount of 2a was increased from 1.5 or 2 equiv. to 3 equiv. Under those conditions, 3aa was obtained in 26% yield (Table 1, entry 12). Again, the addition of silica gel had a positive effect on the yield of 3aa (Table 1, entry 13). Other jar and ball materials affected the product formation as well (Table 1, entries 13–16) with tungsten carbide (WC) being superior over the others (Table 1, entry 16). The same trend was observed when NaOH was replaced by KOH (Table 1, entries 17–20), albeit to a lesser extent. The use of WC proved best leading to 3aa in 57% yield (Table 1, entry 20). Until this stage, 5 equiv. of the base had been added. Increasing the amount of KOH to 6 equiv. had a positive effect on the formation of 3aa, which was now obtained in 85% yield (Table 1, entry 21). Further variations in reaction time, milling frequency, and reagent amounts affected the reaction outcome, but in general, the impact was minor (Table 1, entries 22–27). Thus, the optimal conditions involved the use of 3 equiv. of 2a with respect to 1a, 9 equiv. of S8, 6 equiv. of KOH and SiO2 as grinding auxiliary to be ground in a mixer mill with WC equipment at a frequency of 25 Hz for 90 min (Table 1, entry 21).
Table 1 Optimisation of the reaction conditionsa
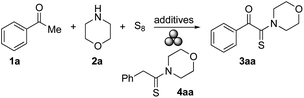
|
Entry |
Jar |
Time (min) |
2a (equiv.) |
Additives (equiv.) |
Yield of 3aa (%) |
Reaction conditions: a mixture of 1a (1 mmol), 2a, S8 (9 mmol), and silica gel (300 mg) were ground in a mixer mill at frequency of 25 Hz at rt in air. The yields refer to isolated amounts of 3aa.
Without grinding auxiliary.
Use of NaSH (2 mmol) instead of S8.
Use of NaCl (300 mg) as grinding auxiliary.
Use of 8 mmol of S8.
Performed at 30 Hz frequency.
|
1b |
SS |
60 |
1.2 |
— |
5 |
2 |
SS |
60 |
1.2 |
— |
10 |
3b |
SS |
60 |
1.2 |
HBF4–SiO2 (0.05) |
9 |
4b |
SS |
60 |
1.5 |
K2CO3 (0.1) |
5 |
5b |
SS |
60 |
1.5 |
p-TSA (0.1) |
Trace |
6b |
SS |
60 |
1.5 |
Na2S (0.1) |
Trace |
7b |
SS |
60 |
1.5 |
CuCl2 (0.1) |
Trace |
8c |
SS |
60 |
1.5 |
— |
Trace |
9 |
SS |
60 |
2 |
I2 (1.5) |
Trace |
10 |
SS |
60 |
2 |
NIS (1.5) |
— |
11b |
SS |
90 |
2 |
— |
5 |
12d |
SS |
90 |
3 |
NaOH (5) |
26 |
13 |
SS |
90 |
3 |
NaOH (5) |
49 |
14 |
PTFE |
90 |
3 |
NaOH (5) |
40 |
15 |
ZrO2 |
90 |
3 |
NaOH (5) |
34 |
16 |
WC |
90 |
3 |
NaOH (5) |
50 |
17 |
SS |
90 |
3 |
KOH (5) |
48 |
18 |
PTFE |
90 |
3 |
KOH (5) |
51 |
19 |
ZrO2 |
90 |
3 |
KOH (5) |
52 |
20 |
WC |
90 |
3 |
KOH (5) |
57 |
21
|
WC
|
90
|
3
|
KOH (6)
|
85
|
22 |
WC |
90 |
3 |
KOH (7) |
82 |
23 |
WC |
90 |
2.5 |
KOH (6) |
72 |
24e |
WC |
90 |
3 |
KOH (6) |
74 |
25 |
WC |
60 |
3 |
KOH (6) |
58 |
26f |
WC |
60 |
3 |
KOH (6) |
59 |
27 |
WC |
120 |
3 |
KOH (6) |
80 |
With the optimized reaction conditions in hand (Table 1, entry 21), several acetophenone derivatives were tested in combination with morpholine (2a) as representative reaction partner. The results are shown in Scheme 2. In general, the reactions proceeded smoothly, providing the corresponding α-ketothioamides (3aa–ma) in moderate to good yields (32–85%). The only exception was p-nitro-substituted acetophenone 1n, which did not give any of the expected α-ketothioamide 3na. A uniform trend on the reaction process and outcome induced by electronic and steric substitution effects could not be identified. Noteworthy, also methyl ketones with heteroarenes reacted well leading to products 3ja–la in yields ranging from 42–73%. Purely aliphatic cyclohexyl methyl ketone (1m) gave α-ketothioamide 3ma in 33% yield.
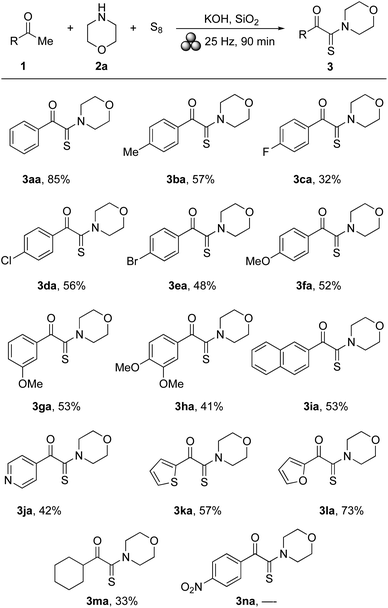 |
| Scheme 2 Scope of acetophenone derivatives. Conditions as described in Table 1, entry 21, yields after column chromatography. | |
Next, the substrate scope with respect to the amine was explored. Acetophenone (1a) served as representative coupling partner. The results are summarised in Scheme 3. Also in these cases, the transformations proceeded well as long as secondary amines were applied. Thus, pyrrolidine (2b), piperidine (2c), and azepane (2d) reacted with 1a to give the corresponding α-ketothioamides 3ab–ad in yields of 68%, 57%, and 49%, respectively. Products 3ae and 3af were obtained from 4-methylpiperidine (2e) and N-methylpiperazine (2f), respectively, and the yields were 61% and 50%. Finally, 1,2,3,4-tetrahydroisoquinoline (2g) afforded 3ag in 49% yield (as a mixture of 2 conformational isomers in a ratio of 2
:
1). Attempts to use secondary (diethyl amine and dibutyl amine) or primary amines (aniline, 4-isopropylaniline, and p-toluidine) remained unsuccessful leading to complex inseparable product mixtures as revealed by TLC.
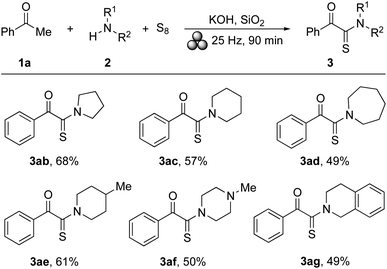 |
| Scheme 3 Scope of cyclic amines. Conditions: Table 1, entry 21; yields after column chromatography. | |
To gain mechanistic insight, several control experiments were conducted, and the results are depicted in Scheme 4.
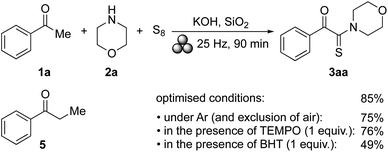 |
| Scheme 4 Control experiments. | |
As described before, the optimised protocol gave 3aa in 85% yield. In order to determine if 3aa was formed via4aa by a break/rebuild mechanism as described by Nguyen and Retailleau (and proposed for the WK reaction),5 the reaction was performed under a strict exclusion of dioxygen. Thus, the reagents were filled into the reaction vial in a glove box, and after filling the container with argon, the jar was tightened with a Teflon tape. As a result, this protocol gave 3aa in 75% yield, suggesting that dioxygen did not play a significant role in the product formation. Experiments performed in the presence of 1 equiv. of TEMPO or BHT gave 3aa in 76% and 49% yield, respectively. Assuming that the latter yield reduction (from the common 85% to 49%) could be attributed to an insufficient mixing of BHT with the other reaction components, we conclude that radicals (including dioxygen) were of low (if any) relevance in the product formation. Furthermore, all attempts to identify 4aa in the product mixture (by MS analysis) failed. Independently prepared 4aa did not give 3aa under the standard conditions. The notion that the formation of 3aa did not follow a standard WK pathway was further supported by the finding that propiophenone (5), which is a commonly well-behaved substrate in WK processes, reacted sluggish under the optimised reaction conditions (Table 1, entry 21) leading to an inseparable mixture of unidentified products (as shown by TLC and NMR analysis). In the absence of sulfur or morpholine, no reaction occurred and acetophenone was the only detectable compound.
Based on these results and in line with a previous suggestion,22 we propose the reaction sequence shown in Scheme 5.
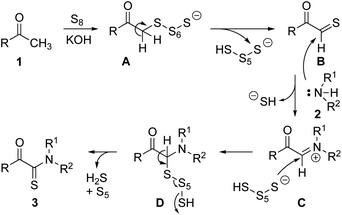 |
| Scheme 5 Proposed mechanistic pathway. | |
Supported by KOH, methyl ketone 1 undergoes smooth keto–enol tautomerisation, and the reaction with sulfur provides intermediate BviaA. When B reacts with amine 2, acyl iminium C is formed, which reacts with an anionic sulfur species providing product 3 after loss of H2S and a shorter sulfur fragment from D.23
Conclusions
In summary, we developed a mechanochemical method for synthesising α-ketothioamides. It is easy to perform, makes use of readily available starting materials, including elemental sulfur, is solvent- and metal-free, and proceeds in short reaction times. The process is reminiscent to solution-based Willgeroth–Kindler reactions, but leads to different products from the same starting materials. Control reactions suggest alternative mechanistic pathways, which do not require dioxygen.
Author contributions
C. C. S. carried out the experiments and data analysis. C. C. S. also wrote the initial draft of the manuscript. S. B. and R. H. helped analysing the data, supported the mechanistic study, and edited the drafts of the manuscript. The project was supervised by C. B., who also provided critical feedback and prepared the final version of the manuscript.
Conflicts of interest
There are no conflicts to declare.
Acknowledgements
R. H. gratefully acknowledges support by the Fonds der Chemischen Industrie e.V. (Kekulé scholarship) and by the Friedrich-Ebert-Stiftung (Promotionsförderung). Mr Nikolaos P. Tripolitsiotis (formerly KU Leuven, Belgium) is thanked for performing initial experiments and for sharing first observations while being at RWTH Aachen University with the support of the Erasmus+ program. All authors thank RWTH Aachen University for general support.
Notes and references
-
(a) K. A. Scott and J. T. Njardarson, Top. Curr. Chem., 2018, 376, 5 CrossRef PubMed;
(b) M. J. Tilby and M. C. Willis, Expert Opin. Drug Discovery, 2021, 16, 1227–1231 CrossRef PubMed;
(c) M. Mustafa and J.-Y. Winum, Expert Opin. Drug Discovery, 2022, 17, 501–512 CrossRef CAS PubMed;
(d) M. Wang and X. Jiang, ACS Sustainable Chem. Eng., 2022, 10, 671–677 CrossRef CAS;
(e) I. Štefanová, P. Bittnerová, J. Zápal, M. Kuzma and R. Kubec, J. Agric. Food Chem., 2023, 71, 5712–5720 CrossRef PubMed.
-
(a) C. Lamberth, J. Sulfur Chem., 2004, 25, 39–62 CrossRef CAS;
(b) C. Lamberth, H. Walter, F. Murphy Kessabi, L. Quaranta, R. Beaudegnies, S. Trah, A. Jeanguenat and F. Cederbaum, Phosphorus, Sulfur Silicon Relat. Elem., 2015, 190, 1225–1235 CrossRef CAS;
(c) J. Yu and X. Jiang, Adv. Agrochem, 2023, 2, 3–14 CrossRef.
-
(a) X. Creary and C. Zhu, J. Am. Chem. Soc., 1995, 117, 5859–5860 CrossRef CAS;
(b) M. A. Ansari, D. Yadav and M. S. Singh, J. Org. Chem., 2020, 85, 8320–8329 CrossRef CAS PubMed;
(c) Z. Zhang, J. Yang, R. Yu, K. Wu, J. Bu, S. Li, P. Qian and L. Sheng, Eur. J. Org Chem., 2021, 5209–5212 CrossRef CAS.
-
(a) S. Ravez, C. Corbet, Q. Spillier, A. Dutu, A. D. Robin, E. Mullarky, L. C. Cantley, O. Feron and R. Frédérick, J. Med. Chem., 2017, 60, 1591–1597 CrossRef CAS PubMed;
(b) Q. Spillier, S. Ravez, J. Unterlass, C. Corbet, C. Degavre, O. Feron and R. Frédérick, Pharmaceuticals, 2020, 13, 20–41 CrossRef CAS PubMed.
- T. B. Nguyen and P. Retailleau, Green Chem., 2017, 19, 5371–5374 RSC.
- For a seminal contribution, see: K. Kindler, Justus Liebigs Ann. Chem., 1923, 431, 187–230 CrossRef CAS.
- Review: D. L. Priebbenow and C. Bolm, Chem. Soc. Rev., 2013, 42, 7870–7880 RSC.
-
(a) T. B. Nguyen, Adv. Synth. Catal., 2017, 359, 1066–1130 CrossRef CAS;
(b) T. B. Nguyen, Adv. Synth. Catal., 2020, 362, 3448–3484 CrossRef CAS;
(c) T. Szabó and M. Milen, Chem. Heterocycl. Compd., 2019, 55, 126–128 CrossRef;
(d) S. Liu, G.-J. Deng and H. Huang, Synlett, 2021, 32, 142–158 CrossRef CAS.
- N.-P. T. Le, T.-H. T. Nguyen, T. K. Nguyen, P.-K. B. Tran and P. H. Tran, J. Chem. Technol. Biotechnol., 2023, 98, 2823–2829 CrossRef CAS.
- T. N. Chaubey, P. J. Borpatra, A. Sharma and S. K. Pandey, Org. Lett., 2022, 24, 8062–8066 CrossRef CAS PubMed.
- J. Dong, C. Sheng, Y. Chen, C. Ni and Y. Wang, Tetrahedron Lett., 2023, 115, 154317 CrossRef CAS.
-
(a) P. Yu, Y. Wang, Z. Zeng and Y. Chen, J. Org. Chem., 2019, 84, 14883–14891 CrossRef CAS PubMed;
(b) L. Gan, Y. Gao, L. Wei and J.-P. Wan, J. Org. Chem., 2019, 84, 1064–1069 CrossRef CAS PubMed;
(c) H.-Z. Li, W.-J. Xue and A.-X. Wu, Tetrahedron, 2014, 70, 4645–4651 CrossRef CAS;
(d) B. Eftekhari-Sis, S. V. Khajeh and O. Büyükgüngör, Synlett, 2013, 977–980 CrossRef CAS.
-
(a) I. d'Anciåes, A. Silva, E. Bartalucci, C. Bolm and T. Wiegand, Adv. Mater., 2023, 2304092, DOI:10.1022/adma.202304092;
(b) E. Juaristi and C. G. Avila-Ortiz, Synthesis, 2023, 55, 2439–2459 CrossRef CAS;
(c) M. T. J. Williams, L. C. Morrill and D. L. Browne, ChemSusChem, 2022, 15, e202102157 CrossRef CAS PubMed;
(d) O. Bento, F. Luttringer, T. M. El Dine, N. Pétry, X. Bantreil and F. Lamaty, Eur. J. Org Chem., 2022, e202101516 CrossRef CAS;
(e) D. Virieux, F. Delogu, A. Procheddu, F. Garcìa and E. Colacino, J. Org. Chem., 2021, 86, 13885–13894 CrossRef CAS PubMed;
(f) T. Friščić, C. Mottillo and H. M. Titi, Angew. Chem., Int. Ed., 2020, 59, 1018–1029 CrossRef PubMed;
(g) S. Mateti, M. Mathesh, Z. Liu, T. Tao, T. Ramireddy, A. M. Glushenkov, W. Yang and Y. I. Chen, Chem. Commun., 2021, 57, 1080–1092 RSC;
(h) A. Porcheddu, E. Colacino, L. de Luca and F. Delogu, ACS Catal., 2020, 10, 8344–8394 CrossRef CAS;
(i) M. Pérez-Venegas and E. Juaristi, ACS Sustainable Chem. Eng., 2020, 8, 8881–8893 CrossRef;
(j) W. Pickhardt, S. Grätz and L. Borchardt, Chem.–Eur. J., 2020, 26, 12903–12911 CrossRef CAS PubMed;
(k) K. Kubota and H. Ito, Trends Chem., 2020, 2, 1066–1081 CrossRef CAS;
(l) I. N. Egorov, S. Santra, D. S. Kopchuk, I. S. Kovalev, G. V. Zyryanov, A. Majee, B. C. Ranu, V. L. Rusinov and O. N. Chupakhin, Green Chem., 2020, 22, 302–315 RSC;
(m) C. Bolm and J. G. Hernández, Angew. Chem., Int. Ed., 2019, 58, 3285–3299 CrossRef CAS PubMed;
(n) E. Colacino, A. Porcheddu, C. Charnay and F. Delogu, React. Chem. Eng., 2019, 4, 1179–1188 RSC;
(o) J. L. Howard, Q. Cao and D. L. Browne, Chem. Sci., 2018, 9, 3080–3094 RSC;
(p) J. G. Hernández and C. Bolm, ChemSusChem, 2018, 11, 1410–1420 CrossRef PubMed;
(q) D. Tan and T. Friščić, Eur. J. Org Chem., 2018, 18–33 CrossRef CAS;
(r) J. G. Hernández and C. Bolm, J. Org. Chem., 2017, 82, 4007–4019 CrossRef PubMed;
(s) J. L. Do and T. Friscic, ACS Cent. Sci., 2017, 3, 13–19 CrossRef CAS PubMed;
(t) D. Tan, L. Loots and T. Friščić, Chem. Commun., 2016, 52, 7760–7781 RSC;
(u) S. L. James, C. J. Adams, C. Bolm, D. Braga, P. Collier, T. Friščić, F. Grepioni, K. D. M. Harris, G. Hyett, W. Jones, A. Krebs, J. Mack, L. Maini, A. G. Orpen, I. P. Parkin, W. C. Shearouse, J. W. Steed and D. C. Waddell, Chem. Soc. Rev., 2012, 41, 413–447 RSC;
(v) A. Bruckmann, A. Krebs and C. Bolm, Green Chem., 2008, 10, 1131–1141 RSC;
(w) B. Rodriguez, T. Rantanen, A. Bruckmann and C. Bolm, Adv. Synth. Catal., 2007, 349, 2213–2233 CrossRef CAS.
-
(a) F. Gomollón-Bel, Chem. Int., 2019, 41, 12–17 CrossRef;
(b) K. J. Ardila-Fierro and J. G. Hernández, ChemSusChem, 2021, 14, 2145–2162 CrossRef CAS PubMed.
- For the use of S8 in Gewald-type reactions, see: W. C. Shearouse, M. Z. Shumba and J. Mack, Appl. Sci., 2014, 4, 171–179 CrossRef.
- For representative examples of mechanochemical reactions with S8 in other fields, see:
(a) W. Yuan, J. Li, Q. Zhang and F. Saito, Powder Technol., 2012, 230, 63–66 CrossRef CAS;
(b) R. Borges, A. R. Sotiles, A. S. Giroto, G. G. F. Guimarães, F. Wypych, N. D. Jablonowski and C. Ribeiro, ACS Agric. Sci. Technol., 2022, 2, 1292–1299 CrossRef CAS;
(c) P. Yan, W. Zhao, F. McBride, D. Cai, J. Dale, V. Hanna and T. Hasell, Nat. Commun., 2022, 13, 4824 CrossRef CAS PubMed.
- For a review focusing on the mechanochemical synthesis of organosulfur heterocycles, see: T. Chatterjee and B. C. Ranu, J. Org. Chem., 2021, 86, 13895–13910 CrossRef CAS PubMed.
- M. D. Goodwin, M. Q. Costa, J. R. Robinson and C. M. Kotyk, Results Chem., 2022, 4, 100528 CrossRef CAS.
-
(a) J. E. Valdez-Rojas, H. Ríos-Guerra, A. L. Ramírez-Sánchez, G. García-González, C. Álvarez-Toledano, J. G. López-Cortés, R. A. Toscano and J. G. Penieres-Carrillo, Can. J. Chem., 2012, 90, 567–573 CrossRef CAS;
(b) W.-W. Liu, Y.-Q. Zhao, R.-B. Xu, L.-J. Tang and H.-W. Hu, Chin. J. Chem., 2006, 24, 1472–1475 CrossRef CAS.
- For an application of HBF4–SiO2 in a setting as in Table 1, entry 3, see: B. P. Bandgar, S. S. Gawande, S. C. Warangkar and J. V. Totre, Bioorg. Med. Chem., 2010, 18, 3618–3624 CrossRef CAS PubMed.
-
(a) M. L. N. Rao and D. N. Jadhav, Tetrahedron Lett., 2006, 47, 6883–6886 CrossRef CAS ; reviews: ;
(b) M. J. Mphahlele, J. Chem. Res., 2010, 34, 121–126 CrossRef;
(c) N. Ajvazi and S. Stavber, Compounds, 2022, 2, 3–24 CrossRef CAS.
- U. C. Kasséhin, F. A. Gbaguidi, C. N. Kapanda, C. R. McCurdy and J. H. Poupaert, Org. Chem. Int., 2014, 486540 Search PubMed.
- For reactions between thioaldehydes (including those with α-carbonyl groups) and elemental sulfur providing thioamides, see: K. Okuma, Y. Komiya and H. Ohta, Bull. Chem. Soc. Jpn., 1991, 64, 2402–2406 CrossRef CAS.
|
This journal is © The Royal Society of Chemistry 2024 |
Click here to see how this site uses Cookies. View our privacy policy here.