DOI:
10.1039/D3RA06931A
(Review Article)
RSC Adv., 2024,
14, 6917-6929
Recent reports on the sensing strategy and the On-site detection of illegal drugs
Received
11th October 2023
, Accepted 13th December 2023
First published on 26th February 2024
Abstract
In this review, works on the on-site detection of illegal drugs in recent years are summarised and discussed, most of which were published within the past five years. The detection methods are categorised as colourimetric, fluorescence, Raman spectrometry, ion mobility spectrometry, electrochemistry, and mass spectrometry. Also, strategies that are possibly suitable for on-site detection and the actual instrumentation to be used in the field are listed.
1. Introduction
Illegal drug abuse is the recreational use of narcotic or psychoactive substances, which are commonly addictive, hallucinogenic, and harmful. The main types of illegal drugs can be divided into two categories: the extracted type including opium, morphine, cannabis, and cocaine; and the synthetic kind, which are mainly based on amphetamines. The amphetamine types could be listed as amphetamine (AMP), methamphetamine (MAMP), 3,4-methylenedioxymethamphetamine (MDMA), and 3,4-methylenedioxyamphetamine (MDA) in detail. Nearly every country prohibits illegal drug abuse due to the extreme harm to human bodies, families, and society. The war against drugs is fierce, and police forces around the world are in great need of drug-detection methods to guide the investigation and conviction of drug dealers. Currently, the detection of drugs in analytical laboratories is mainly based on chromatography,1–3 mass spectrometry,4–7 Raman spectrometry,8–18 and immunoassay.7,19–24
An important detection scenario is detection at an actual crime site by policemen in the field without needing complicated training. A typical strategy for the on-site detection of drugs may follow the principles of colourimetry, electrochemistry, or immunoassay, including time-resolved fluorescence immunoassay (TRFIA), or molecular spectroscopy (Raman spectrometry, ion mobility spectrometry).8,24–27 Compared with traditional chromatography, the spectroscopic method is non-toxic and environmentally friendly, and is thus being increasingly applied in the on-site detection. The Scientific Working Group had provided recommendations for the Analysis of Seized Drugs (SWGDRUG) based on the analytical standards of drugs. Three categories (A, B, and C) of detection strategies were defined based on their discriminating ability.28 Category C covers relatively weak discrimination strategies, including colourimetry, immunoassays, and ultraviolet spectroscopy. Conversely, high specific discrimination relies on Category A techniques, like Raman spectroscopy and mass spectrometry. In actual forensic laboratories, technicians combine Category B as gas chromatography-mass spectrometry (GC-MS) to obtain confirmatory results to support a conviction in court.4–6,29,30 The samples collected on-site typically include captured suspicious powders, bulks, plants, and biological samples such as urine, saliva, and hair. The urine test is a time-saving option, while blood tests require only a drop of peripheral finger blood and are easy to perform. A saliva test has a higher sensitivity than the urine test. Generally, the presence of drugs can be detected in saliva within one month after drug-taking. Hair is also easy to obtain, stable, and certain drugs have a long retention time in hair. However, the drug content in hair is typically low and requires high sensitivity of the detection method.
In this review, works on the on-site detection of illegal drugs in recent years are summarised and discussed, most of which were published within the past five years. Methods are categorised as colourimetric, fluorescence, Raman spectrometry, ion mobility spectrometry, electrochemistry, and mass spectrometry. Strategies possibly suitable for on-site detection and the actual instrumentation to be used in the field are all also listed.
2. Colourimetric methods
Colourimetry is the most common method used for on-site illegal drug detection since the 19th century, when colour and precipitation tests were vital to early forensic toxicological studies to identify plant alkaloids.28 Colourimetry is often determined by colour changes produced in combination with certain chemicals (metal ions, organic solvents, or aromatic compounds) or biological agents (viruses, bacteria. or proteins). Biosensors can be formed by binding specific antibodies or ligands to the target molecule and then using enzyme-labelled secondary antibodies or secondary ligands. Combining these methods with deep UV spectroscopy can provide more comprehensive spectral information in some cases, while still maintaining the quantitative measurement capability of the colourimetric method. After better understanding the chemical structures of plant alkaloids, researchers discovered more and more new colour tests, such as Chen's test for ephedrine31 and Scott's cocaine test.32 With the advent of synthetic drug abuse, more colour testing methods were developed and further applied. Works based on similar principles published by scientists from analytical chemistry and forensic science further provide a wealth of information about colour identification methods for different drugs. From those fundamental research studies, documents, technical instructions, and standards detailing the procedures for colour testing for substance abuse have been issued by various governments.
2.1. Chromogenic reactions
Sensors based on chromogenic reactions use the direct colour change from the drug reaction with test agents or the crystalline state change of products above to identify the presence of the drug. For instance, Lim et al.33 constructed drug screening kits (DSKs) based on the conformation change colour of the conjugate plane (alkene–alkyne backbone) of a polydiacetylene (PDA) sensor when externally stimulated. Reading with the naked eyes is the most simple and convenient approach, but often suffers from a lower accuracy. In the information era, digitised images can be easily obtained, analysed, and quantified by measuring the diffuse reflectance or colour intensity with a mobile phone or other more specialised handheld devices. Generally, a low detection limit of 2–5 g mL−1 and relative standard deviation <10% can be achieved with satisfactory results. Drug-detection kits based on the principle of colourimetry have the characteristics of simple use, rapid detection, portability, excellent sensor stability, and low cost, which makes them suitable for the field screening of drugs.34
The use of a test paper offers a tool for rough determination of the concentration of a drug by the shade of colour produced. For example, the contents of amphetamine-type compounds, such as AMP, MAMP, MDMA, and MDA, could be identified and determined by a solid colourimetric sensor constructed on organo-/inorganic silica particles (PDMS/TEOS/SiO2) modified by 1,2-naphthoquinone-4-sulfonate (NQS).35 The colour was processed by a digital camera, which makes it quite suitable for analysing street samples.
Smartphones nowadays have enough computing power and popularity to support the hardware foundations for in-field detection applications. There have been a series of related works and applications on drug detection using smartphones. For instance, Krauss developed a rotation-driven microfluidic device and a smartphone as a potential alternative for the current colourimetric testing of drugs, enabling objective and humanised image analysis techniques for detection.36 The isolated microfluidic platform could realise the hypothesis testing of drugs in multiple reaction chambers at the same time, to achieve rapid screening.14,15 Smartphones can help identify colour changes objectively. In one study, the hue and saturation image analysis parameters were used to define the threshold values for cocaine and MAMP detection, and the compositions of 30 unknown samples were thereby successfully identified, proving the effectiveness of the method37 (Fig. 1).
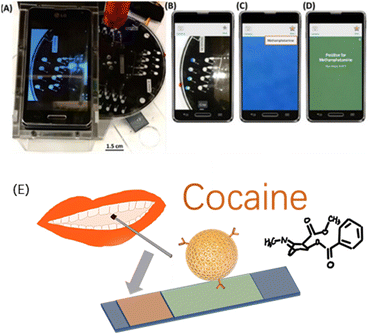 |
| Fig. 1 (A) Image of a drug-detection device, showing the spin system with a PMMA base, PMMA stand and smartphone for imaging, and laser (in red) positioned above the device. (B) Image captured by the device using a smartphone camera. (C) Cropped colour change analysed using a custom application. (D) Results reported qualitatively for a particular drug using defined thresholds, methamphetamine here. Reprinted from ref. 37, with permission. (E) Non-invasive optical sensor for the detection of cocaine and methamphetamine in saliva using rhodamine B-labelled polymersomes ((E) was adapted and redrawn from ref. 38). Reprinted (adapted) with permission from Anal. Chem., 2016, 88(17), 8689–8697. Copyright 2016 American Chemical Society. | |
Hichem et al.38 proposed two colorimetric lateral chromatographic immunoassays (LFIAs) for cocaine (COC) and methamphetamine (METH). In their study, they constructed a non-invasive optical sensor using rhodamine B-labelled polymer bodies to detect cocaine and methamphetamine in saliva.
For the requirements of efficiency and cost for in-field detection, a compact and integrated device for detection and a cooperated sample holder could be developed specifically to a particular detection strategy. Da Silva proposed a colouration device based on office paper, as a fast and low-cost portable sensor designed to detect the adulteration of phenacetin in seized illegal compounds, such as cocaine. White office printer paper was used as the substrate material, and wax-coated printing technology was used to manufacture the test zone. The reaction of NQS and the amino group was still utilised as the chromogenic basis. Under the optimal conditions, it was found that there was a linear relationship between the concentration of phenacetin and the magenta percentage colour, with a limit of detection (LOD) of 3.5 g mL−1. Krauss proposed a simple manufacturing technique to produce a capillary ampoule containing a small amount of chemical agents, which was compatible with a cost-effective thermoplastic centrifugal microfluidic device for use in place of paper materials, while the corrosion resistance of the glass could extend the range of drugs that could be tested to include cocaine, MAMP, MDMA, and MDA.36
2.2. Biosensors
Biosensors work on various biological receptors (enzymes, antibodies, antigens, nucleic acids, etc.), which convert the information of the detection target into signals (electrochemistry, optics, nanomechanics, mass sensitivity, etc.).39 Biosensors have great potential for rapid field sample testing because they are easy to miniaturise and can be used to detect complex targets with minimal sample preparation.40
Due to the specificity, low detection limit, and good versatility of DNA nucleic acid aptamer, drug sensors have been designed based on nucleic acid aptamers. Aptamers bind and form a complex with the target, like cocaine. The binding would initiate conformation change of the aptamer. By careful design of the signal transmission system, the chemical signal indicating the target existence or density can be converted into other detectable optical or electrical signals. Precious metal plasma contributes as a good platform for signal transmission from its low cost and facile application pattern. Yang et al. developed a new colourimetric biosensor for MAMP and cocaine based on Au@Ag core–shell nanoparticles, which offered an excellent logarithm linear correlation of MAMP in the concentration range from 0.5 ppb to 40 ppb, with an LOD of 0.16 ppb.41
Enzyme-based biosensors can also be applied in drug detection. The enzyme-linked immunosorbent assay (ELISA) is the most widely studied and applied technique, due to its high sensitivity and specificity, and low LOD even as low as 1 ng mL−1. Gold colloid particles are also commonly used in combination with ELISA to transmit signals. However, some ELISA kits suffer from low repeatability and a low detection accuracy. At present, the rapid screening of drug addicts, like by MAMP in urine, is based on an ELISA mechanism, but still has limitation in terms of the diversity of the detection targets, the universality of the pretreatment procedure, and inaccuracy or false positives.42 Luo et al. fabricated an enzyme-based system based on a cooperative binding split aptamer (CSBA), also named as CSBAzymes, which formed the keystone to enable the naked-eye detection of cocaine (Fig. 2). CBSAzymes consist of two chains of DNA that are initially separated but can be effectively assembled with the addition of the cocaine target to form a complex. The complex could catalyse the oxidation of 2,2′-azino-bis(3-ethylbenzthiazoline)-6-sulfonic acid (ABTS) into a dark green product. Reportedly, the construction of this sensing CSBAzyme-based system was simple and generalised, while the sensing function was highly sensitive and specific. The strategy itself could also be easily extended for the visual detection of various important small molecules in the future.43 Adegoke reported a novel fluorescent hybrid peroxidase-like catalytic colourimetric sensor based on a nanozyme. The nanozyme system was set up with the combination of quantum dots (L-cysteine-capped ZnSeS), and CTAB-modified AuNPs. Under the optimal catalytic conditions, a specific positive blue-green colour for cocaine identification was obtained within 2 min by a colourimetric method.44
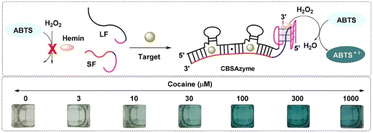 |
| Fig. 2 Working mechanism and the chromogenic catalysis performance of the CSBAzyme. Reprint from ref. 43, with permission. Reprinted (adapted) with permission from Anal. Chem., 2019, 91(11), 7199–7207. Copyright 2019 American Chemical Society. | |
2.3. Deep ultraviolet-visible light spectrometry
Deep ultraviolet-visible (DUV-Vis) spectrometry is derived from conventional UV-Vis spectrometry. However, the conventional UV-Vis method requires at least 100 μL of sample and the corresponding sample tank needs to be carried, which leads to the standard process of dilution of the solution and the need for cleaning of the sample tank. In DUV-Vis spectrometry, more convenient sample handling is possible. Huang et al. constructed a reflective fibre optic sensor to simplify the spectrometric scanning of the absorption peaks of various drugs (Fig. 3). In their optical fibre sensor, a miniatured sample space was constructed between the upper surface and the plane mirror of the optical fibre. The liquid sample was compressed into droplets and immobilised in the sample space by the surface tension of the two surfaces. The DUV-Vis irradiation was provided by a deuterium lamp, transmitting through a central optical fibre and a sample droplet, reflected by the plane mirror, and then transmitted through the sample again. The spectra were analysed and the signals output by software. The absorbance of the sample could then be calculated. By using information of the different spectral absorption peaks of different drugs in the DUV band, the identification and quantitative analysis of various common drugs could be realised quickly, sensitively, and accurately.45 Also, through a new design of the sample holding space, the required sample size was reduced to 0.032–0.16 μL for the detection of common illicit drugs. The sensitivity and resolution were also improved, and the LODs were 0.641, 1.173, 0.447, 0.227, 0.437, and 0.750 μg mL−1 for MDMA, KET hydrochloride, cocaine hydrochloride, diazepam, phenobarbital, and barbiturate, respectively. As an important complement to traditional methods, optical fibre sensors provide a rapid and low-cost in situ micro-sample-amount detection strategy, which could have a great impact on meeting the on-site detection demand (Table 1).
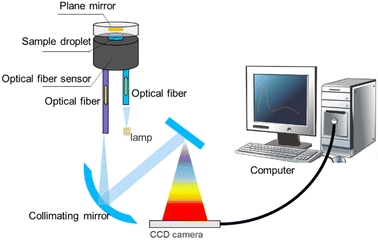 |
| Fig. 3 Diagram of a portable deep ultraviolet-visible spectrophotometric system. | |
Table 1 Performance of some colourimetric-based illicit drug sensors to date
Detection method |
LOD |
Target |
Sample type |
Chromogenic reactions |
0.5 μg μL−1 |
MDMA |
Actual sample |
Chromogenic reactions |
440 pM |
COC |
Prepare sample |
Chromogenic reactions |
2–5 g mL−1 |
COC |
Prepare sample |
Chromogenic reactions |
— |
AMP, MAMP, MDMA, MDA |
Actual sample |
Chromogenic reactions |
0.25 and 0.75 mg mL−1 |
COC and MAMP |
Prepare sample |
Chromogenic reactions |
0.49 ng mL−1 (COC) and 0.37 ng mL−1 (METH) |
COC and METH) |
Actual sample |
Chromogenic reactions |
3.5 g mL−1 |
COC |
Actual sample |
Chromogenic reactions |
0.75 mg mL−1 |
COC, MAMP, MDMA and MDA |
Prepare sample |
Biosensors |
1 ng mL−1 |
MAMP |
Actual sample |
Biosensors |
10 μM |
COC |
Prepare sample |
Deep ultraviolet-visible light spectrometry |
0.641–1.173 μg mL−1 |
MDMA, KET, COC, Diazepam, phenobarbital, and barbiturates |
Prepare sample |
3. Sensing based on fluorescence
Compared with conventional colourimetric analysis, fluorescence detection provides higher sensitivity and objective interpretation of the results.46 Walczak reported a new, highly sensitive image-based fluorescence detection technique instead of the usual colourimetric technique for cocaine (Fig. 4). This technology was integrated into an innovative handheld instrument and side-flow test strip to further develop a diagnostic analysis system on a skin patch for forensic applications.47
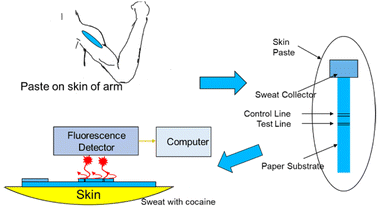 |
| Fig. 4 Schematic visualisation of the side-flow test strip with a sample pad and a handheld fluorescence reader as the first approach for developing a skin patch for the in situ point-of-care monitoring of cocaine abuse. | |
Considering that drugs are usually stored and circulate in the form of salt and have low reactivity and volatility, solid fluorescence sensing faces great challenges.48,49 Nevertheless, a solid-state fluorescent probe does not require any pretreatment for analysis39 or have the disadvantages of existing solution probes. Also, after contact with different concentrations of drugs, emitted light will show different emission colours, which can be seen by the naked eye.48 At present, researchers have developed an unlabelled proportional fluorescence aptamer sensor for the rapid and sensitive detection of cocaine in complex biofluids.40,50,51 Under optimal conditions, the adaptor sensor demonstrated good performance in a linear range of 0.10–10 μM, LOD of 56 nM, and rapid response time within 20 s. The LOD was comparable to that of most fluorescence adaptor sensors with signal amplification arrangements and was much lower than that of all unamplified cocaine aptamer sensors to date. The actual sample analysis of a series of complex biofluids, such as urine, saliva and serum, also showed that the solid fluorescence sensor had good precision, stability, and high sensitivity, indicating its great potential for the on-site screening of cocaine in complex biofluids.27 He et al. reported the first paper device based on upper-conversion nanoparticles (UCNPs) for the roadside field testing of cocaine.27 Once two properly designed aptamer fragments identified the cocaine, the luminescence from the nanoparticles immobilised on the paper was quenched by Au nanoparticles (AuNPs), also indicating the cocaine concentration.52 A simple smartphone could be utilised to calculate quantitative results in a short time with high sensitivity. Besides, the device was suitable for human saliva samples and blood samples.
4. Raman spectrometry
Raman spectroscopy and near-infrared (NIR) spectroscopy are two of the most widely used techniques in forensic detection chemistry. When combined with stoichiometric tools, they can build very powerful analytical platforms with a fingerprint library of related compounds.9,12,53–55 Also, advances in the instrumentation for Raman and NIR analyses have facilitated a miniaturisation of the common desktop devices for field detection applications. Raman technology includes Raman spectroscopy and surface-enhanced Raman spectroscopy (SERS), which can even enable the sensitive detection of fentanyl in heroin samples.8,56 Raman technology application in drug screening offers high sensitivity, rapidness, and non-contact, non-destructive characteristics.
Raman spectroscopy detection methods are widely used in drugs analysis and the development of new techniques, including near-infrared laser Raman spectroscopy (NIRS),57,58 Raman confocal microscopy,59 and spatially offset Raman spectroscopy (SORS). Near-infrared dispersive Raman spectroscopy has the advantages of portability, a short analysis time, and wide application range. Its most attractive capability is it enables direct detection through containers to effectively avoid pollution. Confocal microscopy Raman combines Raman spectroscopy and microscopic analysis to for high resolution. Different from the backscattering in traditional Raman spectroscopy, which can only detect ∼100 μm shallow surface of a sample, SORS technology can analyse opaque samples to several millimetres depth. The key principle of the method is to collect and analyse the Raman signal from a certain displacement of the laser spot.
Most synthetic drugs, such as MDMA, MAMP, ketamine (KET), ephedrine (EPH), and methephedrine, can be detected by Raman spectroscopy. In the field application of Raman techniques for drug detection, the intensity of Raman scattering is always very weak, and it is challenging at the trace amount level. SERS addresses this shortcoming by generating a strong signal enhancement from closeness to the metal nanoparticles.8–18,41,53,56,60–62 Lombardi et al. studied a kind of paper-based material immersed with silver nanoparticles, which was used to detect trace amounts of drugs, such as fentanyl (Fig. 5).8 Yang et al. used liquid–liquid microextraction/SERS10,53 and a liquidphase interface nanoparticle array coupled with SERS18 to determine morphine in human urine.
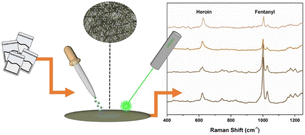 |
| Fig. 5 Use of Raman spectroscopy as an effective tool for detecting illicit drugs. Reprinted from ref. 8 with permission. Reprinted (adapted) with permission from Anal. Chem., 2018, 90(21), 12678–12685. Copyright 2018 American Chemical Society. | |
To overcome the need for large and expensive laboratory equipment and enable SERS to be applied for on-site detection, the combination of SERS and a microfluidic platform is an option, and offers a way to overcome other barriers too, such as a lack of reproducibility when using inexpensive substrates for high sensitivity. Based on this idea, several examples of portable drug testing have been reported.11,13–15,17,23,55,56,63 However, to bring more SERS portable devices to the market to realise their considerable market potential for field application, three significant difficulties must be overcome for rapid detection: the rapid separation and enrichment of the drug molecules; interference from complex components in urine; the SERS-active nanostructure hot spot efficiency. A portable kit was developed to solve these problems, and to realise the rapid detection of the molecular fingerprint characteristics of drugs with the use of highly reproducible SERS substrates (Fig. 6). The kit could simultaneously detect and identify various drugs in human urine. The kit comprised a set of 3 min sample pretreatment materials, a standardised substrate for a highly repeatable point array, and a handheld Raman device for SERS analysis. The LOD was a low 0.1 ppm, which was sufficient for law enforcement to perform the rapid detection of METH, MDMA, and other common drugs in urine.64
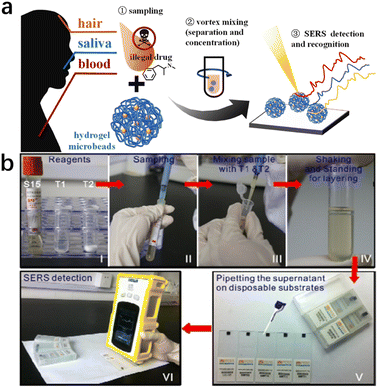 |
| Fig. 6 (a) Pretreatment-free, on-site separation and sensitive identification of methamphetamine in biological specimens by SERS-active hydrogel microbeads.65 (b) Illustration of a portable kit for the rapid SERS detection of drugs in real human urine. Reprinted from ref. 64 with permission. Reprinted (adapted) with permission from Zhenzhen Han et al. Anal Chem., 2015. Copyright 2015 American Chemical Society. Copyright 2023 Elsevier B.V. | |
Ye Z. et al.65 prepared pore-selective SERS-active hydrogel microbeads, with Ag nanoparticles uniformly dispersed and wrapped in the hydrogel matrix, which showed excellent SERS performance. By using these SERS hydrogel microbeads, methamphetamine (MAMP) could be rapidly and reliably detected in various biological specimens (blood, saliva, and hair) without sample pretreatment (Fig. 7).
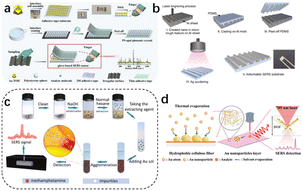 |
| Fig. 7 (a) Flexible and wearable glove-based SERS sensor for the rapid sampling and sensitive detection of controlled drugs. (b) Development of a cost-effective fabrication process for on-site methamphetamine detection by an adsorbable SERS substrate. (c) Ultrafast and field-based detection of methamphetamine in hair by Au nanocake-enhanced Raman spectroscopy. (d) Direct thermal growth of gold nanopearls on 3D interwoven hydrophobic fibres as ultrasensitive portable SERS substrates for clinical applications.67–69 Reprinted (adapted) with permission from Han Z. Portable kit for the identification and detection of drugs in human urine using surface-enhanced Raman spectroscopy. Anal Chem., 2015, 87(18), 9500–9506. Copyright 2015 American Chemical Society. Copyright 2022 Elsevier B.V. Copyright 2023 Wiley-VCH GmbH. Copyright 2022 Elsevier B.V. | |
Recently, more attention has been paid to flexible wearable in situ detection methods based on SERS. Maneejark et al.66 developed a cost-effective fabrication process for on-site methamphetamine detection using an adsorbable SERS substrate. Li et al.67 used gold nanocake-enhanced Raman spectroscopy for the ultrafast and field detection of methamphetamine in hair; while M. Zhang et al.68 developed a wearable flexible glove surface-enhanced Raman scattering (SERS) sensor for the field detection of tramadol and midazolam and the accurate identification of methamphetamine; and Wan et al.69 prepared a wafer-grade, ultrasensitive, highly homogeneous, paper-based, portable SERS detection platform. The latter platform had an extremely high Raman enhancement factor of 3.9 × 1011, subfemtomolar concentration detection capability (down to the single molecule level), and excellent signal reproducibility (relative standard deviation of 3.97%).
The combination of Raman spectroscopy and IMS technology and the fusion of innovative data of two spectrograms could improve the drug detection. The results showed that the coupling strategy could also achieve an effective improvement of the speed and accuracy compared to any single system alone, thus further providing a safe, rapid, and reliable new analytical method for drug identification (Table 2).55,61,70
Table 2 Data from research studies on illicit drug sensors based on fluorescence and Raman spectroscopy
Detection method |
LOD |
Target |
Sample type |
Sensing based on fluorescence |
1 ng mL−1 |
COC |
Actual sample |
Sensing based on fluorescence |
56 nM |
COC |
Actual sample |
Raman spectrometry |
3 μg L−1 |
Fentanyl, morphine |
Actual sample |
Raman spectrometry |
0.1 ppm |
MA, MDMA and MC |
Actual sample |
Raman spectrometry |
0.1 ppm |
MAMP |
Actual sample |
Raman spectrometry |
— |
MAMP |
Actual sample |
Raman spectrometry |
69.19 ng mL−1 and 35.03 ng mL−1 |
Tramadol and midazolam, AMP |
Actual sample |
5. Electrochemistry
The principle of the electrochemical method is to analyse components based on the relationship between electrical parameters (voltage, conductance, current, and electric quantity, etc.) and the information of the analyte according to the electrochemical properties of substances in solution. MAMP and heroin can be detected by electrochemical oxidation. A typical microfluidic electrochemical sensing set-up that has potential for on-site application is shown in Fig. 8. The electrochemical method has some key advantages, including high sensitivity, high specificity, wide measuring range, low cost, and portability of the measuring instruments and equipment. It is highly suitable for quick on-site testing. However, electrochemical methods also have some drawbacks. The electrode operation (usually based on a three-electrode set-up) requires frequent calibration resulting from its poor stability because of electrode poisoning/fouling problems.71
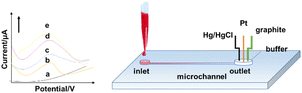 |
| Fig. 8 Schematic of drug detection by an electrochemical method. | |
The electrochemical oxidation method can be used to detect amphetamine class drugs (such as MDMA) with an LOD as low as 2.4 μM in serum in a three-electrode system by determination of the oxidation peak appearing in the linear scan diagram.72 Similar methods have also been applied for detecting cocaine,73 THC,74 and MAMP.75 Dronova et al. first utilized electrochemical sensing for cannabinoids synthesized from new psychoactive substances with the trade name of “spice”.71 Electrooxidation tests of 11 new synthetic cannabinoids with an indole substructure, representing the main components in mixtures that are illegally smoked in the drug market, were performed by cyclic and differential pulse voltammetry. The study found that the synthetic cannabinoids showed a detectable voltammetric response in the smoking mixtures and artificial saliva, which was limited to the nM scale. Voltammetry thus provides a tool for pre-screening synthetic cannabinoid derivatives from seized materials and biological samples.
5.1. Electrochemistry based on the molecular imprint
Molecular imprinting can be combined with electrochemistry to form new detection methods. Akhoundian et al. reported a voltammetric strategy combined with a molecularly imprinted polymer (MIP) for the trace level sensing of MAMP in human urine and serum. The reported LOD was 0.83 ppb, which was the lowest LOD ever reported. This strategy was based on the fast Fourier-transform square-wave voltammetric (FFT-SWV) method at an MIP/multi-walled carbon nanotube (MWCNTs)-modified carbon paste electrode.76 For the precursors of MAMP, like N-formylamphetamine, a capacitive sensing approach was developed by Beloglazova with a similar PMAA-MIP strategy. In this case, the LOD was 10 μM when using a MIP-immobilized gold electrode.77
In addition, Li Niu et al.78 demonstrated an efficient electrochemical surface plasmon resonance (EC-SPR) sensor combined with a molecular imprinting strategy for the adsorption and quantitative measurement of ATS in human urine and serum samples. Using 3,4-methylenedioxyphenylethylamine (MDEA) as the template molecule and dopamine (DA) as the functional monomer, a molecular imprint recognition system on an SPR chip was synthesized using a convenient one-step electrochemical polymerization method. In particular, the molecular imprinting process could be regulated and controlled by simultaneously measuring the SPR signals. The detection limits of this method for 3,4-methylenedioxyamphetamine (MDA) and 3,4-methylenedioxymethamphetamine (MDMA) were 3 nM and 4 nM, respectively, while the linear range was quite wide and resistant to interference from a variety of illegal drugs and other substances (Fig. 9).
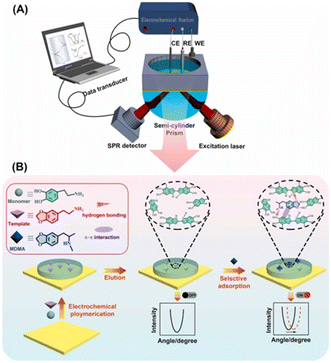 |
| Fig. 9 Novel electrochemical surface plasmon resonance (EC-SPR) sensor for the detection of amphetamine-type stimulants based on a molecularly imprinted strategy. (A) Schematic diagram of the equipment; (B) Mechanism diagram of the mechanism of action.76–78 Copyright 2017 Elsevier B.V. Copyright 2019 Elsevier B.V. | |
5.2. Electrochemical biosensors
In the conversion of a chemical signal to an electrochemical signal, the change in electrochemical signal before and after the aptamer DNA binds with the target analyte can be used to detect the target analyte, and plays an important role in rapid screening. With the appearance of synthetic oligonucleotides, the functional electrochemical platform can be with fixed with antibodies or aptamers to make sensors for the detection of biological signal together with possible signal amplification for detection of the target analytes. The aptamer can also be combined with electrochemical luminescence (ECL) technology, which can utilize electrochemical reactions to produce directly observable results. To detect drugs like cocaine through optical signal conversion, a highly sensitive and reusable ECL sensor was prepared based on the double-covalent-bond immobilisation method of click chemistry with a diazo moiety79 (Fig. 10). In this approach, the cocaine aptamer acted as the molecular recognition component, while the ruthenium/pyridine derivatives offered the ECL signal. In the presence of cocaine, the increase in ECL signals showed a good linear relationship with the concentration of cocaine in the range of 0.1–100 nM, and the LOD was 60 pM. The sensor demonstrated good stability and could be used repeatedly.
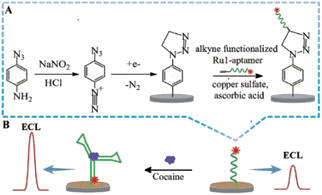 |
| Fig. 10 Novel electrochemical-surface plasmon resonance (EC-SPR) sensor for the detection of amphetamine-type stimulants based on a molecularly imprinted strategy: (A) schematic diagram of the equipment; (B) mechanism of action. Copyright 2022 Elsevier B.V. | |
5.3. Wearable sensors based on electrochemistry
In recent years, due to their low cost, strong flexibility, and miniaturisation potential, great attention has been paid to the coupling production of wearable devices with electrochemistry sensors.80 For instance, flexible electrochemical biosensors based on nitrile butadiene rubber gloves with a highly stretchable printed electrode system were developed as a wearable screening tool for national defence and food safety applications.81 These disposable, mechanically robust “lab on gloves” integrated stretchable, printable enzyme-based biosensors and moving surfaces that could be wiped and sampled on different fingers, combined with a compact electronic interface for electrochemical detection and real-time wireless data transmission to smartphone devices. Also, Wang et al. developed a wearable glove-based sensor that could electrochemically detect fentanyl on fingertips (Fig. 11). The flexible screen-printed carbon electrode was the key component for the direct oxidation and subsequent sensing of fentanyl through square-wave voltammetry. Samples could be in a liquid or powder form and the system demonstrated an LOD of 10 μM. An incorporated communication module allowed wirelessly transmitting the results to a smartphone or tablet for further analysis. This all-in-one integrated sampling and sensing platform on the thumb allowed the rapid screening of fentanyl, showing great potential for this technology.42
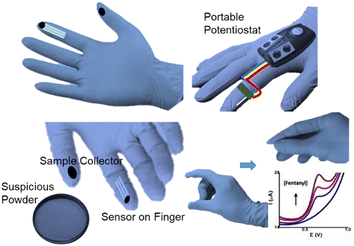 |
| Fig. 11 Lab on a glove system. | |
Wang et al. further described another kind of wearable sensing device in the form of a ring for the detection of salivary THC as well as alcohol. THC and alcohol were detected with voltammetry and amperometry, respectively. The key sensing zone consisted of an MWCNT/carbon electrode for THC, and a Prussian-blue transducer and an alcohol oxidase/chitosan reagent layer for alcohol. The wireless communication module was integrated for use by law enforcement personnel to rapidly screen motorists, achieving results in 3 min per sample (Fig, 12).82
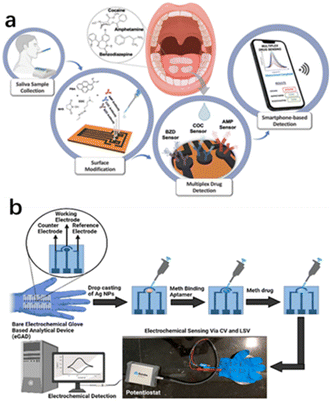 |
| Fig. 12 (a) Smart multiplex point-of-care platform for simultaneous drug monitoring. (b) Wearable electrochemical glove-based analytical device (eGAD) for the detection of methamphetamine employing silver nanoparticles.83,84 Copyright 2015 American Chemical Society. | |
Timur et al.83 developed a multiplex laser-scribed graphene (LSG) sensing platform that could be used for the simultaneous rapid and sensitive electrochemical detection of amphetamine (AMP), cocaine (COC), and benzodiazepines (BZD) in saliva samples. Such a multi-detection sensing system combined with a custom potentiostat enabled the development of a complete point-of-care (POC) platform. Smartphone integration was achieved through customised applications for operating, displaying, and sending data. Similarly, Narang et al.84 proposed a new wearable electrochemical glove analysis device (eGAD) specifically for the detection of methamphetamine. The sensor immobilized methyl aptamers on silver nanoparticles (AgNPs)-modified electrodes printed on latex gloves. The developed sensor had a detection limit of 0.1 μg mL−1, quantitation limit of 0.3 μg mL−1, linear concentration range of approximately 0.01–5 μg mL−1, and recovery rates of approximately 102% and 103%, respectively. The sensor was also valid for 60 days, making it a practical option with a reasonable shelf life (Table 3).
Table 3 Date from electrochemical-based illegal drug sensor research
Detection method |
LOD |
Target |
Sample type |
— |
2.4 μM |
MDMA, COC, THC and MAMP |
Actual sample |
Electrochemistry based on molecular imprinting |
0.83 ppb |
MAMP |
Actual sample |
Electrochemistry based on molecular imprinting |
3 nM, 4 nM |
MDEA |
Actual sample |
Electrochemical biosensors |
60 pM |
COC |
Prepare sample |
Wearable sensor based on electrochemistry |
10 μM |
Fentanyl |
Actual sample |
Wearable sensor based on electrochemistry |
— |
THC and alcohol |
Actual sample |
Wearable sensor based on electrochemistry |
0.1 μg mL−1 |
AMP, COC and BZD |
Actual sample |
6. Mass spectrometry
The new spectroscopy-based technologies mentioned above can meet the requirements for rapid on-site drug detection to a certain extent, but they still have certain limitations. For instance, the sample pretreatment process necessary for using these technologies is usually a multi-step process involving sampling, extraction, enrichment, purification, and derivation. The pretreatment process is also time-consuming and prone to cross-contamination and information errors. Besides, weak spectral signals can be easily affected by other spectra or light environments.85,86 Alternatively, thermal desorption technology is widely used to transfer analytes from the solid or liquid to gas phase, which is an indispensable step in the field detection of drugs. A miniature ion trap mass spectrometer was proposed for the rapid detection of complex matrix drugs in the field.87,88 Polytetrafluoroethylene swabs used in the through-hole gas ionisation method have good water absorption and are suitable for the rapid sampling of any surface drug. Also, solvent-assisted thermal desorption can be used in the test to analyse drugs in saliva.
The combination of environmental ionisation and micro mass spectrometry can be used for field analysis without the need for cumbersome sample pretreatment.89 It thus represent a new technical idea for the rapid field detection of drugs. For example, the direct detection of small molecules, such as drugs, has been achieved in the field by combining desorption electrospray ionisation with a portable mass spectrometer system.90 The Mini 12 system, comprising a desktop micro mass spectrometer with an environmental ionisation source and tandem mass spectrometry capability, has been developed and used for characterisation.91 This device is an independent system for the quantitative analysis of samples, including non-volatile analytes (Fig. 1). Paper spray (PS) is another environmental ionisation method. Zheng et al. developed a method for the rapid monitoring and quantification of drug abuse in dry blood spots using paper spray tandem micro mass spectrometry (PS-MS).92 In their study, 15 μL blood was collected in the form of dry blood spots deposited on a triangular chromatographic paper. A small amount of spray solvent was added to the paper to extract the analyte from the sample. High pressure (∼3500 V) was then applied to the paper to conduct spray ionisation. Drugs in the blood were quantified using tandem mass spectrometry in a multi-response monitoring model (Fig. 13).
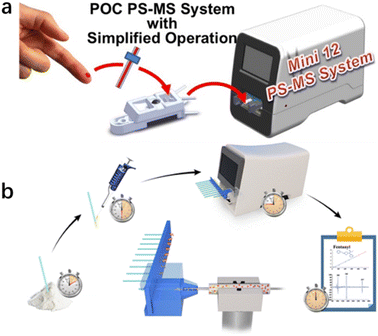 |
| Fig. 13 (a) Mini 12 system that provides a simplified drug-detection operational protocol; (b) detection flow chart.94 Copyright 2014 American Chemical Society. | |
In addition, good progress has been made in the miniaturization of mass spectrometers, especially for use in areas such as environmental monitoring, public safety, and space exploration. Miniature mass spectrometers have also shown great potential for the on-site analysis of illicit drugs when coupled with ambient ionization methods.93 In 2021, matrix-assisted ionization was also coupled to the Mini β system for the suspect screening of fentanyl analogues by Guo et al.94
These studies demonstrated the feasibility of the environmental ionisation combined portable mass spectrometer for use in the field detection of drugs. With the proliferation of new drugs, there is a constant need to develop new and powerful environmental ionisation technologies to expand the range of detection systems for drugs.95
7. Ion mobility spectroscopy (IMS)
Although the colourimetric method has been well developed and even applied for rapid field detection, most tests require a calibration of the colours produced in different light environments and may require certain additional operations, such as the mixing of reagents on site. IMS technology, a drug-detection instrument for ion mobility determination with high sensitivity, can directly detect trace ephedrine, heroin, cocaine, methamphetamine, and other illegal drugs (Fig. 14). As a fast detection technique, ion migration spectroscopy (IMS) has been widely used96–101 to detect illicit drugs. In general, IMS uses thermal desorption to volatilise the collected analyte and then passes this to gas-phase ionization,102 a nickel radioactive source,103 photoionization,104 or corona discharge ionization.105 The separation is carried out according to the electromobility of the drift chamber. The ion trap operation mode is usually adopted, which allows ions to accumulate in the reaction zone before entering the drift zone. This structure eliminates the loss caused by gate electrode cycling. Using IMS technology, the detection sensitivity can reach the ng L−1 level under certain conditions within only a few seconds.97 Those characteristics are very practical in drug detection in the field. Copyright 2018 Elsevier B.V.
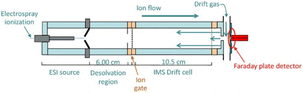 |
| Fig. 14 Schematic cross-sectional view of electrospray ionisation high-performance ambient pressure ion mobility spectrometry. Reprint from ref. 97, with permission. | |
There is already commercially available portable IMS equipment available, and this has been used for fast on-site chemical classification and the identification of tetrahydrocannabinol (THC) varieties and the detection of illegal THC.106 IMS analysis is fast, simple, sensitive, and portable, making it a good option for on-site drug detection.101 IMS combined with thermal desorbed technology can be used to obtain spectral fingerprints of individual cannabinoids from cannabis plant extracts and cannabis plant residues. Other non-cannabis plants and tobacco can also be analysed and classified using this method.100 However, it should also be noted that IMS has some disadvantages, such as poor selectivity and its false positive rate.97 To improve the sensitivity in drug detection and avoid the limitations caused by the mobility range of ion shutters, Chen et al. developed a system with an inner diameter of 14 mm and drift length of 38.9 mm in a minified ion migration spectrometer equipped with a double compression tri-state ion shutter to improve the gated performance. This set-up could greatly reduce the mobility and improve component identification accuracy by compressing the ion packets (Tables 4 and 5)).99
Table 4 Data from research on illicit drug sensors based on IMS and mass spectrometry
Detection method |
LOD |
Target |
Sample type |
Ion mobility spectroscopy (IMS) |
— |
THC |
— |
Mass spectrometry |
— |
Topical drug |
Actual sample |
Mass spectrometry |
270 ng mL−1 |
Drugs in blood |
Actual sample |
Mass spectrometry |
|
Fentanyl analogues |
Actual sample |
Table 5 Comparative analysis of the six methods covered in this study
Method |
Pros |
Cons |
Cost |
Colourimetry |
-Rapid response |
-Lacks reusability |
Low |
-Ease-of-use |
Fluorescence |
-Sensitivity |
-Sensitive to chemical environment |
Low |
-Specificity |
-Life span stability and photo stability |
-Multiple response modes (intensity and decay time) |
-Autofluorescence interference |
-Robust to light scattering |
|
Raman spectrometry |
-Minimum water by effect |
-Test time |
Medium |
-Specificity |
-Specificity |
|
-Unstable |
Electrochemistry |
-Accuracy |
-Life span |
Low |
-Efficiency |
-Sensitive to the environment |
-Portability |
-False positive possibility |
Mass spectrometry (incl. IMS) |
-Reliability |
-Difficulty of quantification |
Medium– high |
-Specificity |
-Sample status versatility |
8. Conclusions
This review summarised the latest reports on the on-site detection strategies of illegal drugs that have been published in the last five years. Almost all the major principles in analytical chemistry have been studied and applied in this subject. Some have even went beyond the results merely in the laboratory and integrated these into devices or sensing toolkits. Operating characteristics like good sensitivity, rapid response, strong anti-interference capability, versatility to handle various sample statuses, and convenience to perform a test in the field are the key goals being pursued by researchers. To meet the best balance of all those merits for detecting different kinds of drugs, blanks in the method vs. the drug matrix should be filled. This means that when some methods or improved strategies have been reported for one kind of target, they should be tried on a similar target for comparison. Such developments also require multidisciplinary considerations of mechanics, optics, and electronic engineering to translate basic analytical chemistry results from the laboratory into system for actual application by law enforcement personnel. Most researchers in this field today are working on the front line to support the control of illegal drugs, especially by the systematic detection of illegal drugs. Regarding a future perspective in this area, we expect that cheaper, more rapid, and reliable methods will be developed that can offer abundant information for various actual application scenarios. As actual users of different methods but not developers of them, we are working towards the combination of two or more strategies by benefitting from their advantages for a particular use. We are also expecting portable apparatuses to be developed with modular and flexible characteristics that could allow a fast response towards newly emerging drugs or psychoactive substances.
Author contributions
Conceptualization: Changxu Lin, Yang Zhao. Funding acquisition: Yang Zhao. Writing – original draft: Yang Zhao. Writing – review & editing: Yang Zhao, Yumeng Gan, Ying Chang, Zheng, Jun Chen, Changxu Lin.
Conflicts of interest
There are no conflicts to declare.
Acknowledgements
This research has been supported by the China National Key R&D Program during the 14th Five-year Plan Period (Grant No. 2021YFF0602501). Technical Research Project funded by the Ministry of Public Security of China (2020JSYJC12)
Notes and references
- T. M. Binz, E. Williner, P. Strajhar, P. C. Dolder, M. E. Liechti, M. R. Baumgartner, T. Kraemer and A. E. Steuer, Drug Test. Anal., 2018, 10, 254–261 CrossRef CAS PubMed.
- F. Been, J. O'Brien, F. Y. Lai, M. Morelato, P. Vallely, J. McGowan, A. L. N. van Nuijs, A. Covaci and J. F. Mueller, Drug Test. Anal., 2018, 10, 1590–1598 CrossRef CAS PubMed.
- C. Cutler and S. Hudson, Drug Test. Anal., 2019, 11, 1134–1143 CrossRef CAS PubMed.
- A. B. Fialkov, S. J. Lehotay and A. Amirav, J. Chromatogr. A, 2019, 460691, DOI:10.1016/j.chroma.2019.460691.
- E. d. S. Santos, E. Spinelli, A. A. Vieira and S. V. Rodrigues, Talanta, 2019, 191, 545–552 CrossRef PubMed.
- A. Song and J. Yang, Anal. Chim. Acta, 2019, 1045, 162–168 CrossRef CAS PubMed.
- N. V. Guteneva, S. L. Znoyko, A. V. Orlov, M. P. Nikitin and P. I. Nikitin, Microchim. Acta, 2019, 186 Search PubMed.
- A. Haddad, M. A. Comanescu, O. Green, T. A. Kubic and J. R. Lombardi, Anal. Chem., 2018, 90, 12678–12685 CrossRef CAS PubMed.
- N. Nuntawong, P. Eiamchai, W. Somrang, S. Denchitcharoen, S. Limwichean, M. Horprathum, V. Patthanasettakul, S. Chaiya, A. Leelapojanaporn, S. Saiseng, P. Pongsethasant and P. Chindaudom, Sensor. Actuator. B Chem., 2017, 239, 139–146 CrossRef CAS.
- B. Yu, M. Ge, P. Li, Q. Xie and L. Yang, Talanta, 2019, 191, 1–10 CrossRef CAS PubMed.
- R. Salemmilani, B. D. Piorek, R. Y. Mirsafavi, A. W. Fountain, III, M. Moskovits and C. D. Meinhart, Anal. Chem., 2018, 90, 7930–7936 CrossRef CAS PubMed.
- C. Li, Y. Qin, D. Li, C. Zhang, A. Liang, G. Wen, Z. Lu and Z. Jiang, Sensor. Actuator. B Chem., 2018, 255, 3464–3471 CrossRef CAS.
- S. Siddhanta, M. S. Wrobel and I. Barman, Chem. Commun., 2016, 52, 9016–9019 RSC.
- X. Kong, X. Chong, K. Squire and A. X. Wang, Sensor. Actuator. B Chem., 2018, 259, 587–595 CrossRef CAS PubMed.
- N. D. Kline, A. Tripathi, R. Mirsafavi, I. Pardoe, M. Moskovits, C. Meinhart, J. A. Guicheteau, S. D. Christesen and A. W. Fountain III, Anal. Chem., 2016, 88, 10513–10522 CrossRef CAS PubMed.
- X. Cao, M. Qin, P. Li, B. Zhou, X. Tang, M. Ge, L. Yang and J. Liu, Sensor. Actuator. B Chem., 2018, 268, 350–358 CrossRef CAS.
- H. Dies, J. Raveendran, C. Escobedo and A. Docoslis, Sensor. Actuator. B Chem., 2018, 257, 382–388 CrossRef CAS.
- Y. Ma, H. Liu, M. Mao, J. Meng, L. Yang and J. Liu, Anal. Chem., 2016, 88, 8145–8151 CrossRef CAS PubMed.
- N. A. Abdelshafi, U. Panne and R. J. Schneider, Talanta, 2017, 165, 619–624 CrossRef CAS PubMed.
- J. Canoura, Z. Wang, H. Yu, O. Alkhamis, F. Fu and Y. Xiao, J. Am. Chem. Soc., 2018, 140, 9961–9971 CrossRef CAS PubMed.
- C. Esen, J. Czulak, T. Cowen, E. Piletska and S. A. Piletsky, Anal. Chem., 2019, 91, 958–964 CrossRef CAS.
- S. van der Heide, A. Cunningham, S. Hardwick and D. A. Russell, Analyst, 2016, 141, 6116–6125 RSC.
- J. C. Vidal, J. R. Bertolin, L. Bonel, L. Asturias, M. Julia Arcos-Martinez and J. R. Castillo, J. Pharm. Biomed. Anal., 2016, 125, 54–61 CrossRef CAS.
- Y. Zhang, Z. Sun, L. Tang, H. Zhang and G.-J. Zhang, Microchim. Acta, 2016, 183, 2791–2797 CrossRef CAS.
- Q. Li, T. Qiu, H. X. Hao, H. Zhou, T. Z. Wang, Y. Zhang, X. Li, G. L. Huang and J. Cheng, Analyst, 2012, 137, 1596–1603 RSC.
- J. N. Scherer, T. R. Fiorentin, B. T. Borille, G. Pasa, T. R. Vieira Sousa, L. von Diemen, R. P. Limberger and F. Pechansky, J. Pharm. Biomed. Anal., 2017, 143, 77–85 CrossRef CAS PubMed.
- M. A. Beatty, A. J. Selinger, Y. Li and F. Hof, J. Am. Chem. Soc., 2019, 141, 16763–16771 CrossRef CAS PubMed.
- M. Philp and S. Fu, Drug Test. Anal., 2018, 10, 95–108 CrossRef CAS PubMed.
- J. F. Casale, E. S. Casale, S. G. Toske, P. A. Hays and S. Panicker, Drug Test. Anal., 2017, 9, 462–469 CrossRef CAS.
- M. Pan, P. Xiang, Z. Yu, Y. Zhao and H. Yan, J. Chromatogr. A, 2019, 1587, 209–226 CrossRef CAS PubMed.
- K. K. Chen, J. Am. Pharmaceut. Assoc., 1929, 18, 110–116 CAS.
- L. Scott Jr, Microgram, 1973, 6, 179–181 Search PubMed.
- S. Jang, S. U. Son, B. Kang, J. Kim, J. Lim, S. Seo, T. Kang, J. Jung, K. S. Lee, H. Kim and E. K. Lim, Anal. Chem., 2022, 94, 3535–3542 CrossRef CAS PubMed.
- K. Abnous, N. M. Danesh, M. Ramezani, S. M. Taghdisi and A. S. Emrani, Anal. Chim. Acta, 2018, 1020, 110–115 CrossRef CAS PubMed.
- A. Argente-Garcia, N. Jornet-Martinez, R. Herraez-Hernandez and P. Campins-Falco, Anal. Chim. Acta, 2016, 943, 123–130 CrossRef CAS PubMed.
- S. T. Krauss, M. S. Woolf, K. C. Hadley, N. M. Collins, A. Q. Nauman and J. P. Landers, Sensor. Actuator. B Chem., 2019, 284, 704–710 CrossRef CAS.
- S. T. Krauss, T. P. Remcho, S. M. Lipes, R. Aranda, H. P. Maynard III, N. Shukla, J. Li, R. E. Tontarski Jr and J. P. Landers, Anal. Chem., 2016, 88, 8689–8697 CrossRef CAS PubMed.
- F. Ghorbanizamani, H. Moulahoum and S. Timur, IEEE Sensor. J., 2022, 22, 1146–1153 CAS.
- S. Rauf, L. Zhang, A. Ali, Y. Liu and J. Li, ACS Sens., 2017, 2, 227–234 CrossRef CAS PubMed.
- K. Mao, Z. Yang, J. Li, X. Zhou, X. Li and J. Hu, Talanta, 2017, 175, 338–346 CrossRef CAS PubMed.
- K. Mao, Z. Zhou, S. Han, X. Zhou, J. Hu, X. Li and Z. Yang, Talanta, 2018, 190, 263–268 CrossRef CAS PubMed.
- A. Barfidokht, R. K. Mishra, R. Seenivasan, S. Liu, L. J. Hubble, J. Wang and D. A. Hall, Sens. Actuators, B, 2019, 296, 126422 CrossRef CAS PubMed.
- Y. Luo, H. Yu, O. Alkhamis, Y. Liu, X. Lou, B. Yu and Y. Xiao, Anal. Chem., 2019, 91, 7199–7207 CrossRef CAS PubMed.
- O. Adegoke, C. McKenzie and N. N. Daeid, Sensor. Actuator. B Chem., 2019, 287, 416–427 CrossRef CAS.
- Q. Li, T. Qiu, H. Hao, H. Zhou, T. Wang, Y. Zhang, X. Li, G. Huang and J. Cheng, Analyst, 2012, 137, 1596–1603 RSC.
- Y. Tang, F. Long, C. Gu, C. Wang, S. Han and M. He, Anal. Chim. Acta, 2016, 933, 182–188 CrossRef CAS PubMed.
- R. Walczak, J. Kruger and S. Moynihan, Meas. Sci. Technol., 2015, 26, 085401 CrossRef.
- T. Fan, W. Xu, J. Yao, Z. Jiao, Y. Fu, D. Zhu, Q. He, H. Cao and J. Cheng, ACS Sens., 2016, 1, 312–317 CrossRef CAS.
- K. Liu, C. Shang, Z. Wang, Y. Qi, R. Miao, K. Liu, T. Liu and Y. Fang, Nat. Commun., 2018, 9, 1695 CrossRef PubMed.
- F. D. Morris, E. M. Peterson, J. M. Heemstra and J. M. Harris, Anal. Chem., 2018, 90, 12964–12970 CrossRef CAS PubMed.
- J. Wang, J. Song, X. Wang, S. Wu, Y. Zhao, P. Luo and C. Meng, Talanta, 2016, 161, 437–442 CrossRef CAS PubMed.
- M. He, Z. Li, Y. Ge and Z. Liu, Anal. Chem., 2016, 88, 1530–1534 CrossRef CAS PubMed.
- B. Yu, C. Cao, P. Li, M. Mao, Q. Xie and L. Yang, Talanta, 2018, 186, 427–432 CrossRef CAS PubMed.
- J. Eliaerts, P. Dardenne, N. Meert, F. Van Durme, N. Samyn, K. Janssens and K. De Wael, Drug Test. Anal., 2017, 9, 1480–1489 CrossRef CAS PubMed.
- W. R. de Araujo, T. M. G. Cardoso, R. G. da Rocha, M. H. P. Santana, R. A. A. Munoz, E. M. Richter, T. Paixao and W. K. T. Coltro, Anal. Chim. Acta, 2018, 1034, 1–21 CrossRef CAS PubMed.
- R. Salemmilani, M. Moskovits and C. D. Meinhart, Analyst, 2019, 144, 3080–3087 RSC.
- C. A. F. O. Penido, M. T. T. Pacheco, R. A. Zângaro and L. Silveira Jr, J. Forensic Sci., 2015, 60, 171–178 CrossRef CAS PubMed.
- K. Tsujikawa, T. Yamamuro, K. Kuwayama, T. Kanamori, Y. T. Iwata, K. Miyamoto, F. Kasuya and H. Inoue, J. Forensic Sci., 2016, 61, 1208–1214 CrossRef CAS PubMed.
- C. A. F. de Oliveira Penido, M. T. T. Pacheco, I. K. Lednev and L. Silveira Jr, J. Raman Spectrosc., 2016, 47, 28–38 CrossRef.
- L. Xiao, R. Alder, M. Mehta, N. Krayem, B. Cavasinni, S. Laracy, S. Cameron and S. Fu, Drug Test. Anal., 2018, 10, 761–767 CrossRef CAS PubMed.
- H. Segawa, T. Fukuoka, T. Itoh, Y. Imai, Y. T. Iwata, T. Yamamuro, K. Kuwayama, K. Tsujikawa, T. Kanamori and H. Inoue, Analyst, 2019, 144, 2158–2165 RSC.
- J. Meng, X. Tang, B. Zhou, Q. Xie and L. Yang, Talanta, 2017, 164, 693–699 CrossRef CAS PubMed.
- G. O. da Silva, W. R. de Araujo and T. R. L. C. Paixao, Talanta, 2018, 176, 674–678 CrossRef CAS PubMed.
- Z. Han, H. Liu, J. Meng, L. Yang, J. Liu and J. Liu, Anal. Chem., 2015, 87, 9500–9506 CrossRef CAS PubMed.
- Z. Ye, H. Yao, Y. Zhang, A. Su, D. Sun, Y. Ye, J. Zhou and S. Xu, Anal. Chim. Acta, 2023, 1263, 341285 CrossRef CAS PubMed.
- K. Maneejark, N. Sangwaranatee, N. Chamchoi, N. Kulnides, P. Somboonsaksri, S. Limwichean, T. Pogfay, S. Kalasung, P. Eiamchai, V. Patthanasettakul, N. Limsuwan, N. Triamnak, N. Nuntawong and M. Horprathum, Opt. Mater., 2022, 124 Search PubMed.
- W. Peng, X.-Q. Zong, T.-T. Xie, J.-W. Zhou, M.-F. Yue, B.-Y. Wen, Y.-H. Wang, J. Chen, Y.-J. Zhang and J.-F. Li, Anal. Chim. Acta, 2022, 1235, 340531 CrossRef CAS PubMed.
- M. Zhang, Y. Lu, L. Zhang, X. Xu, B. Li, X. Zhao, X. Yan, C. Wang, P. Sun, X. Liu and G. Lu, Sensor. Actuator. B Chem., 2023, 386 Search PubMed.
- H. Y. Lin, W. R. Chen, L. C. Lu, H. L. Chen, Y. H. Chen, M. Pan, C. C. Chen, C. Chen, T. H. Yen and D. Wan, Small, 2023, 19, e2207404 CrossRef PubMed.
- L. Harper, J. Powell and E. M. Pijl, Harm Reduct. J., 2017, 14, 52 CrossRef PubMed.
- M. Dronova, E. Smolianitski and O. Lev, Anal. Chem., 2016, 88, 4487–4494 CrossRef CAS PubMed.
- E. M. P. J. Garrido, J. M. P. J. Garrido, N. Milhazes, F. Borges and A. M. Oliveira-Brett, Bioelectrochemistry, 2010, 79, 77–83 CrossRef CAS PubMed.
- J. M. Freitas, D. L. O. Ramos, R. M. F. Sousa, T. R. L. C. Paixão, M. H. P. Santana, R. A. A. Muñoz and E. M. Richter, Sensor. Actuator. B Chem., 2017, 243, 557–565 CrossRef CAS.
- M. Renaud-Young, R. M. Mayall, V. Salehi, M. Goledzinowski, F. J. E. Comeau, J. L. MacCallum and V. I. Birss, Electrochim. Acta, 2019, 307, 351–359 CrossRef CAS.
- Ľ. Švorc, M. Vojs, P. Michniak, M. Marton, M. Rievaj and D. Bustin, J. Electroanal. Chem., 2014, 717–718, 34–40 CrossRef.
- M. Akhoundian, T. Alizadeh, M. R. Ganjali and P. Norouzi, Talanta, 2019, 200, 115–123 CrossRef CAS PubMed.
- K. Graniczkowska, M. Pütz, F. M. Hauser, S. De Saeger and N. V. Beloglazova, Biosens. Bioelectron., 2017, 92, 741–747 CrossRef CAS PubMed.
- C. Li, D. Han, Z. Liang, F. Han, W. Fu, W. Wang, D. Han, Y. Wang and L. Niu, Sensor. Actuator. B Chem., 2022, 369 Search PubMed.
- Q. Hong-lan, W. Bing, W. Xiao-fei, Z. Cheng-xiao and Z. Ting, J. Electrochem., 2019, 25, 223–231 Search PubMed.
- M. S. Mahmud, H. Fang, S. Carreiro, H. Wang and E. W. Boyer, Smart Health, 2019, 13, 100062 CrossRef.
- R. K. Mishra, L. J. Hubble, A. Martin, R. Kumar, A. Barfidokht, J. Y. Kim, M. M. Musameh, I. L. Kyratzis and J. Wang, ACS Sens., 2017, 2, 553–561 CrossRef CAS PubMed.
- R. K. Mishra, J. R. Sempionatto, Z. Li, C. Brown, N. M. Galdino, R. Shah, S. Liu, L. J. Hubble, K. Bagot, S. Tapert and J. Wang, Talanta, 2020, 211, 120757 CrossRef CAS PubMed.
- D. Beduk, T. Beduk, J. I. de Oliveira Filho, A. Ait Lahcen, E. Aldemir, E. Guler Celik, K. N. Salama and S. Timur, ACS Appl. Mater. Interfaces, 2023, 15, 37247–37258 CrossRef CAS PubMed.
- N. Anzar, S. Suleman, Y. Singh, S. Parvez, M. Khanuja, R. Pilloton and J. Narang, Biosensors, 2023, 13 Search PubMed.
- J. Sanchez-Gonzalez, S. Garcia-Carballal, P. Cabarcos, M. Jesus Tabernero, P. Bermejo-Barrera and A. Moreda-Pineiro, J. Chromatogr. A, 2016, 1451, 15–22 CrossRef CAS PubMed.
- D. V. M. Sousa, F. V. Pereira, C. C. Nascentes, J. S. Moreira, V. H. M. Boratto and R. M. Orlando, Talanta, 2020, 208 Search PubMed.
- W. Wang, C. Xu, H. Ruan, H. Li, Y. Xing, K. Hou and H. Li, Anal. Methods, 2020, 12, 264–271 RSC.
- S. Wang, W. M. Wang, H. Li, Y. M. Xing, K. Y. Hou and H. Y. Li, Anal. Chem., 2019, 91, 3845–3851 CrossRef CAS PubMed.
- X. X. Ma and Z. Ouyang, Trac. Trends Anal. Chem., 2016, 85, 10–19 CrossRef CAS PubMed.
- T. J. Kauppila, N. Talaty, T. Kuuranne, T. Kotiaho, R. Kostiainen and R. G. Cooks, Analyst, 2007, 132, 868–875 RSC.
- L. F. Li, T. C. Chen, Y. Ren, P. I. Hendricks, R. G. Cooks and Z. Ouyang, Anal. Chem., 2014, 86, 2909–2916 CrossRef CAS PubMed.
- Y. Su, H. Wang, J. J. Liu, P. Wei, R. G. Cooks and Z. Ouyang, Analyst, 2013, 138, 4443–4447 RSC.
- Y. Zhai, X. Fu and W. Xu, Mass Spectrom. Rev., 2023, 1–20 Search PubMed.
- X. Guo, Y. Shang, Y. Lv, H. Bai and Q. Ma, Anal. Chem., 2021, 93, 10152–10159 CrossRef CAS PubMed.
- J. H. Kennedy, J. Palaty, C. G. Gill and J. M. Wiseman, Rapid Commun. Mass Spectrom., 2018, 32, 1280–1286 CrossRef CAS PubMed.
- Z. Du, T. Sun, J. Zhao, D. Wang, Z. Zhang and W. Yu, Talanta, 2018, 184, 65–72 CrossRef CAS PubMed.
- A. B. Kanu and A. Leal, Anal. Chem., 2016, 88, 3058–3066 CrossRef CAS PubMed.
- T. P. Forbes and M. Najarro, Analyst, 2016, 141, 4438–4446 RSC.
- H. Chen, C. Chen, W. Huang, M. Li, Y. Xiao, D. Jiang and H. Li, Anal. Chem., 2019, 91, 9138–9146 CrossRef CAS PubMed.
- M. d. M. Contreras, N. Jurado-Campos, C. Sanchez-Carnerero Callado, N. Arroyo-Manzanares, L. Fernandez, S. Casano, S. Marco, L. Arce and C. Ferreiro-Vera, Sensor. Actuator. B Chem., 2018, 273, 1413–1424 CrossRef CAS.
- D. J. Cocovi-Solberg, F. A. Esteve-Turrillas, S. Armenta, M. de la Guardia and M. Miro, J. Chromatogr. A, 2017, 1512, 43–50 CrossRef CAS PubMed.
- T. Porta, E. Varesio and G. Hopfgartner, Anal. Chem., 2013, 85, 11771–11779 CrossRef CAS PubMed.
- S. Wilkinson, B. Muir and J. Coumbaros, in Encyclopedia of Forensic Sciences, ed. J. A. Siegel, P. J. Saukko and M. M. Houck, Academic Press, Waltham, 2nd edn, 2013, pp. 641–645, DOI:10.1016/B978-0-12-382165-2.00258-0.
- C. Chen, D. Jiang and H. Li, Anal. Chim. Acta, 2019, 1077, 1–13 CrossRef CAS PubMed.
- M. Behpour, S. Nojavan and Z. Ghaniyari, Int. J. Ion Mobil. Spectrom., 2020, 23, 167–175 CrossRef.
- S. Armenta, M. Alcala and M. Blanco, Anal. Chim. Acta, 2011, 703, 114–123 CrossRef CAS PubMed.
Footnote |
† Co-first author. |
|
This journal is © The Royal Society of Chemistry 2024 |
Click here to see how this site uses Cookies. View our privacy policy here.