DOI:
10.1039/D4SC05081A
(Edge Article)
Chem. Sci., 2024,
15, 16196-16204
A-site cation manipulation of exemplary second harmonic generation response and optical anisotropy in rare-earth borates†
Received
30th July 2024
, Accepted 6th September 2024
First published on 6th September 2024
Abstract
Ultraviolet nonlinear optical (UV NLO) materials have garnered significant interest for their prospective applications in advanced laser technologies. However, tailoring the desired structure in these materials remains a formidable challenge. Here, we propose a simple yet effective strategy for synthesizing rare-earth borates, KxNa3−xLa2B3O9 (x = 2–3), by manipulating the A-site cations to induce structural evolution. Notably, KxNa3−xLa2B3O9 undergoes a phase transition from the Pnc2 to the Amm2 space group by adjusting the K+ content to reach x = 2.6. Moreover, the target compounds exhibit strong phase-matching second harmonic generation (SHG) efficiencies, ranging from 1.3 to 3.3 times that of KDP (KH2PO4), and feature short UV cutoff edges of around 204–208 nm. Additionally, the correlation between microscopic polarizability, optical anisotropy, and the structural evolution of these materials was characterized through structural and theoretical analyses. These findings highlight the potential applications of KxNa3−xLa2B3O9 as UV NLO materials and underscore the viability of manipulating A-site cations to fabricate NLO crystals with desirable properties.
Introduction
Nonlinear optical (NLO) crystals have garnered considerable attention in scientific and industrial applications due to their ability to manipulate laser frequency and produce coherent light. In particular, ultraviolet (UV) NLO materials have emerged as crucial contenders for extending the wavelength range of solid-state lasers into the UV region, facilitated by a second harmonic generation (SHG) process.1–3 These materials hold significant promise in various optoelectronic fields such as laser processing, short-wave communication, semiconductor lithography, biomedicine, and high-density storage.4–7 Despite considerable efforts and advancements in exploring acentric materials, the practical realization of UV NLO crystals remains limited. Noteworthy examples include KH2PO4 (KDP), β-BaB2O4 (β-BBO), LiB3O5 (LBO), KBe2BO3F2 (KBBF), and CsLiB6O10 (CLBO), among others.8–12 In response to the development of laser technology, there is an increasing demand for novel NLO crystals capable of directly generating UV coherent light. However, the fabrication of NLO crystals faces challenges stemming from the inherent propensity of inorganic materials to exhibit undesired dipole–dipole interactions or spatial effects, tending to crystallize centrosymmetric structures.13,14 Consequently, the development of innovative synthetic strategies to exploit NLO crystals with SHG-enhanced activity remains a significant challenge.
Borates, characterized by their versatile structural configurations and favorable UV transparency attributed to strong covalent B–O bonds, have emerged as a candidate system for exploring UV optical crystals.15–19 To effectively innovate and construct novel NLO crystals, the introduction of highly polarizable chromophores into borates is an optional strategy to modify the structure and enhance the optical properties. Strategies such as incorporating second-order Jahn–Teller (SOJT) active cations (Zr4+, Nb5+, Ta4+, etc.), stereo-chemically active lone-pair cations (Te4+, Bi3+, etc.), or d10 transition metal cations (Zn2+, Cd2+, etc.) have been widely recognized in the quest for new optical materials.20–22 Following these design strategies, numerous borates with acentric structures have been synthesized, including BaZr(BO3)2, CsNbB2O6, K3M3B2O12 (M = Nb, Ta), α-BiB3O6, CaBi2B2O7, Cd4BiO(BO3)3, Bi3TeBO9, Na2ZnB6O11, Cs3Zn6B9O21, and CaZn2(BO3)2.2,23–31 However, the strategies for excavating UV NLO crystals are still limited due to challenges in accurately regulating the structure.
Recent studies have elucidated the indispensable role of alkali metal cations (A-site cations) in the exploration of novel NLO materials, due to the fact that alkali metal cations are devoid of d–d or f–f electron transitions, enabling the broadening of the bandgap and consequently achieving short absorption edges.32 Additionally, alkali metal cations with various ionic radii also have a significant impact on the spatial molecular arrangements and macroscopic symmetry. Notable examples include RbB4O6F (0.8 × KDP), CsB4O6F (1.9 × KDP), CsRbB8O12F2 (1.1 × KDP), K7SrY2B15O30 (1.1 × KDP), Rb7SrY2(B5O10)3 (0.9 × KDP), Na3La2(BO3)3 (2 × KDP), and KNa2La2(BO3)3 (2.6 × KDP),33–38 exhibiting different NLO activities attributed to the presence of diverse A-site cations. A similar situation has also been observed in alkali metal borophosphate and phosphate UV NLO crystals, such as Rb3B11P2O23 (2.5× KDP), Cs3B11P2O23 (3 × KDP), α-KZnPO4 (0.2 × KDP) and α-LiZnPO4 (2.3 × KDP).39–41 These investigations suggest that the optimization of local structures induced by A-site cations may enhance SHG performance. However, limited research has been conducted thus far on the evolution process of the structure and performance of distinct A-site cations in these NLO borate-based derivatives.
To tackle this challenge, we utilized rare earth borates as a foundation and introduced alkali metals with varying ionic radii to optimize the structural arrangement and optical properties by adjusting the composition of alkali metals. For instance, the substitution of Na+ and K+ cations is widely regarded as a viable approach due to the inherent flexibility in doping between these components.42 Additionally, the incorporation of rare earth cations, like Sc3+, Y3+, La3+, Gd3+ and Lu3+, not only contributes to excellent transparency in the UV region, but also offers various coordination types.43 Notably, the La3+ cation with a sizable ionic radius tends to form flexible SHG-active chromophores, thereby generating favorable SHG effects.38 Inspired by these ideas, our work focuses on rare earth borate-based materials and explores the underlying mechanism of the NLO effect and optical anisotropy by employing a facile strategy to manipulate the components of A-site alkali metal cations. The synthesis of rare-earth borates KxNa3−xLa2B3O9 (x = 2–3) was achieved through structural evolutions attributed to A-site cation manipulations. As anticipated, these compounds displayed remarkable phase-matching SHG efficiencies ranging from 1.3 to 3.3 times that of KDP, while featuring short UV cutoff edges of approximately 204 nm. These findings highlight the potential of KxNa3−xLa2B3O9 as favorable candidates for UV NLO applications and underscore the significance of regulating A-site alkali metal cations in the exploration of new NLO crystals.
Results and discussion
Synthesis and phase transformation
The preparation of the target compounds was performed through a modified solid-phase reaction. To monitor the influence of A-site cations on the crystal structure, a series of compounds with varying proportions of A-site cations, namely K2, K2.2, K2.4, K2.6, K2.8, and K3, were synthesized. The phase purity of the resulting products was verified using PXRD analysis. It is evident from Fig. 1a that the K2 and K3 compounds demonstrate distinct crystal structural characteristics. Specifically, the structural characteristics of K2.8 and K3 exhibited isomorphism, suggesting a consistent structural framework despite the varying proportions of K cations in the A-site. However, when the K+ cation proportion reached approximately K2.6, structural features indicative of the K2 compound were initially observed, implying the coexistence of two distinct polycrystalline phases. This observation confirms that the structural transition from K3 to K2 occurs at around the K2.6 proportion. Furthermore, the PXRD peak patterns of K2, K2.2, and K2.4 displayed a high degree of similarity, suggesting isomorphism between these compounds. These observations provide clear evidence of the critical impact of the A-site cation proportions on the structural regulation of these materials. Notably, as illustrated in Fig. 1b, an increase in the K proportion within the range of K2–K2.4 leads to a gradual shift of PXRD peaks towards lower angles, indicating larger lattice constants.44 This trend is also observed when considering the proportion of K variation within the K2.8–K3 range. These findings strongly suggest that the proportion of K+ cations exerts a direct impact on the lattice constants of these compounds.
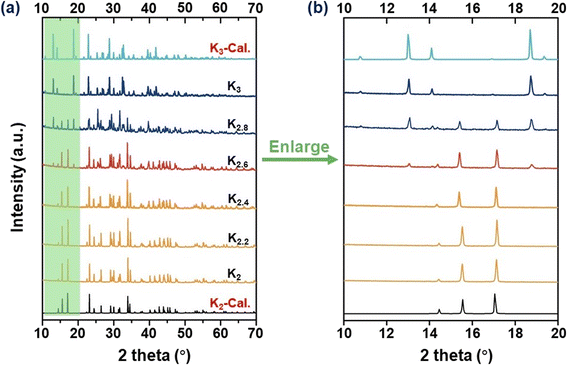 |
| Fig. 1 (a) Experimental and calculated PXRD patterns for K2, K2.2, K2.4, K2.6, K2.8, and K3 polycrystals, respectively. (b) The enlarged PXRD curves observed in the 2θ range of 10–20°. | |
Thermal analyses
Thermal analyses were conducted to further investigate the structural transformations and evaluate the thermal stability of the title compounds. TG-DTA measurements revealed the remarkable thermal stability of the K2, K2.2, K2.4, K2.6, K2.8, and K3 compounds, as evidenced by sharp absorption peaks observed above 850 °C (Fig. 2a). Notably, the thermal stability of compounds within the K2–K2.6 range exhibited a decreasing trend with increasing proportions of K+ cations (Fig. 2b). In contrast, within the K2.6–K3 range, the compounds displayed an upward trend in thermal stability (Fig. 2g). Among the tested compound systems, it has been observed that the K2.6 compound demonstrated the lowest thermal stability. When the K+ cation reaches a composition of 2.6, this behavior can potentially be attributed to the influence of mixed components present in the system that cannot be ignored, leading to a decrease in the melting point of the system. These findings further highlight the role of the K2.6 compound, which possesses mixed-phase structures that align with the PXRD analyses.
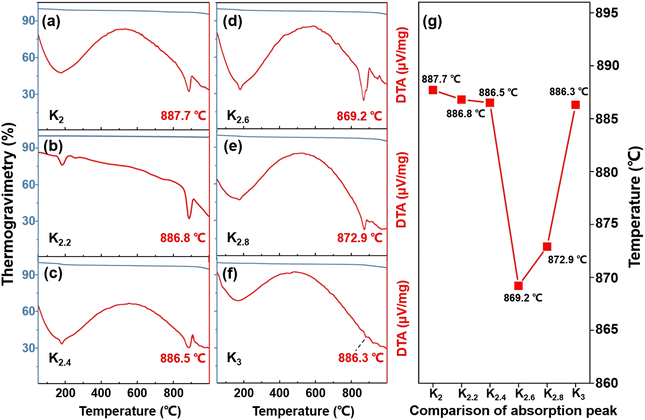 |
| Fig. 2 (a–f) TG-DTA curves for the K2, K2.2, K2.4, K2.6, K2.8, and K3 compounds, respectively. (g) Comparative results of thermal stabilities for the title compounds. | |
Crystal structures of K2 and K3
In light of the isomorphism observed among the K2, K2.2, and K2.4 compounds, as well as the isomorphism between the K2.8 and K3 compounds, we have selected K2 and K3 as representative samples for conducting a thorough analysis of their respective crystal structures. The structural features of K2 and K3 were determined through the utilization of single-crystal XRD analysis (Tables S1–S5†). Our analysis revealed that K2 adopts an asymmetric orthorhombic crystal structure, belonging to the space group Amm2 (no. 38), while K3 exhibits a similar orthorhombic structure in the Pnc2 space group (no. 30), as summarized in Table S1.† In the crystal structure of K2, the asymmetric unit consists of two distinct K, one Na, one La, two B, and four O atoms. Conversely, the asymmetric unit of K3 contains three K, three La, four B, and eleven O atoms. As shown in Fig. 3a and b, the B atoms are coordinated by three O atoms, forming [BO3] plane triangles, and the La atom is surrounded by nine O atoms, resulting in the formation of distorted [LaO9] polyhedra. The B–O bond lengths in K2 range from 1.360(2) to 1.379(12) Å, while the La–O bond lengths range from 2.466(12) to 2.687(5) Å. In K3, the B–O bond lengths vary from 1.300(5) to 1.409(15) Å, whereas the La–O bond lengths range from 2.412(8) to 3.02(2) Å. In K2, adjacent [LaO9] polyhedra are connected to each other through oxygen edge-sharing, forming a pseudo-one-dimensional (1D) chain denoted as [La2O16]∞. These 1D chains, along with [BO3] units, further interconnect through oxygen corner-sharing to form pseudo-two-dimensional (2D) layers on the bc plane (Fig. 3c). These pseudo-2D layers are then bridged with [BO3] units along the a-axis through oxygen corner-sharing, ultimately giving rise to a three-dimensional (3D) structural framework (Fig. 3d). In the case of K3, three distorted [LaO9] polyhedra associate to form distinct [La3O21] clusters via oxygen face-sharing. These clusters are further interconnected with [BO3] units by sharing oxygen along the bc plane, as presented in Fig. 3e, with the K+ cations located in the channels (Fig. 3f).
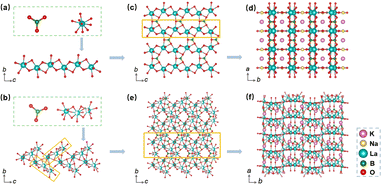 |
| Fig. 3 Structural characteristics of K2 and K3. (a and b) [BO3] plane triangles, [LaO9] polyhedra, and [La2O16]∞ and [La3O21] clusters. (c) Pseudo-2D layers of K2. (d) 3D structural framework of K2. (e) Presentation of [La3O21]∞ clusters connected with [BO3] units in K3 viewed from the bc plane. (f) 3D structural network of K3 viewed along the c-axis. | |
To shed light on the structural evolution exhibited by the K2 and K3 compounds, our study focuses on a thorough analysis of the arrangement and interrelationships of fundamental [BO3] units. Notably, while both K2 and K3 compounds feature isolated planar triangles formed by [BO3] units, a notable disparity arises in the spatial arrangement of these triangles within their respective 3D frameworks. As shown in Fig. 4a–c, it is noteworthy that the two distinct [BO3] plane triangles in K2 exhibit a regular arrangement, manifesting a constant angle of 90 degrees between the planes they occupy. In contrast, the planes defined by the distinct [BO3] units in K3 feature varying angles, including 63.312, 89.061, and 69.942 degrees, which deviate significantly from the uniform 90 degree arrangement observed in K2. The variations in the spatial angles between the [BO3] building blocks in K2 and K3 have distinct implications for the anisotropic characteristics of their respective structures. These observations would contribute to a more comprehensive understanding of the potential structural and application-related implications associated with the different spatial arrangements of [BO3] units in K2 and K3.
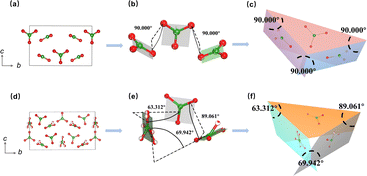 |
| Fig. 4 Comparison of the arrangements of distinct [BO3] units in the K2 and K3 compounds. (a–c) Arrangements and orientations of the isolated [BO3] units in the K2 compound. (d–f) Arrangements and orientations of the isolated [BO3] units in the K3 compound. | |
Spectroscopic properties
To investigate the optical properties of the title compounds, the UV-vis-NIR diffuse reflectance spectra of the title compounds were recorded under consistent conditions (Fig. 5). The obtained spectra revealed distinct UV transmittance cut-off edges for each compound: 208, 206, 206, 207, 208, and 204 nm for the K2, K2.2, K2.4, K2.6, K2.8, and K3 compounds, respectively. These favorable UV transmittance characteristics directly correspond to energy band gaps of 5.15, 5.24, 5.20, 5.19, 5.23, and 5.29 eV, respectively, as determined using the Kubelka–Munk formula.45 Furthermore, we conducted a detailed analysis of the infrared (IR) spectra of the compounds, which revealed similar characteristics among them, as depicted in Fig. S1.† Taking the K2 compound as an example, the absorption peaks observed at around 1392.73 and 1200.69 cm−1 are attributed to the asymmetric stretching vibrations of the [BO3] units, while the peak near 950.08 cm−1 corresponds to the symmetric stretching vibrations. The peaks around 743.24 and 604.83 cm−1 are caused by the bending vibrations of the [BO3] units. The presence of these distinct vibration modes further confirms the existence of the [BO3] unit within the compounds.37,38,43b Notably, we observed a slight redshift phenomenon in the absorption peak positions of the IR spectrum with an increasing K to Na component ratio. This trend can primarily be attributed to the expansion of the alkali metal cation radius.46 With a larger cation radius, the bond length between the cation and oxygen atom increases, resulting in a lower vibration frequency and a consequent redshift in the absorption peak position of the IR absorption spectrum. These findings provide valuable insights into the intrinsic relationship between structural changes and the optical properties exhibited by the investigated compounds.
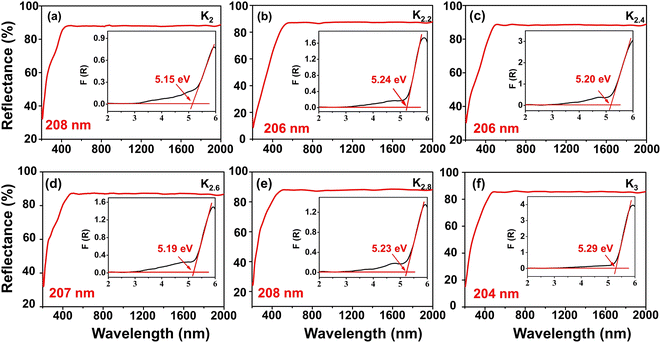 |
| Fig. 5 (a–f) UV-vis-NIR diffuse reflectance spectra for the K2, K2.2, K2.4, K2.6, K2.8, and K3 polycrystalline samples, respectively. | |
SHG characterization
The title compounds are considered to possess SHG responses because they crystallize in non-centrosymmetric space groups. Fig. 6a presents a plot illustrating the correlation between the particle size and the detected powder SHG signals. It is observed that the SHG response intensity of each compound increases with increasing particle size until it reaches its maximum value, suggesting phase-matching behavior in accordance with the rules proposed by Kurtz and Perry.47
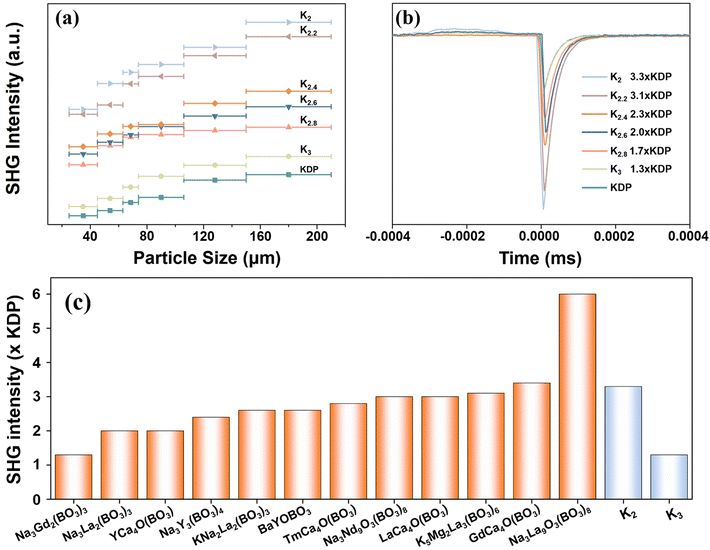 |
| Fig. 6 (a) Phase-matched curves for KDP, K2, K2.2, K2.4, K2.6, K2.8, and K3 polycrystalline powders under 1064 nm laser irradiation. (b) Comparison of SHG intensity between the KDP reference and the title compounds. (c) Comparison of the reported SHG intensity of alkali/alkaline earth metal rare earth borates containing [BO3] units. | |
Furthermore, the SHG intensities of K2, K2.2, K2.4, K2.6, K2.8, and K3 were determined to be 3.3, 3.1, 2.3, 2.0, 1.7, and 1.3 times that of the benchmark KDP, respectively, within a particle size range of 177 to 210 μm (Fig. 6b). The SHG responses are sufficiently large for UV NLO applications. Notably, the significant disparity in the SHG effect between K2 and K3 can primarily be attributed to the disordered arrangement of oxygen atoms in K3, which gives rise to an inconsistent alignment of the [BO3] triangular units, consequently resulting in a lower microscopic second-order hyper-polarizability compared to K2.48 Additionally, as the ratio of K to Na in these compounds gradually increases, the SHG response correspondingly decreases. This observation may be closely related to the unit cell volume of the crystal structure, where a larger unit cell volume tends to weaken the second-order polarizability. Fig. 6c and Table S6† provide a compilation of the reported SHG intensities of alkali and alkaline earth metal borate compounds containing [BO3] triangular units from the literature.49 Notably, K2 exhibits a significant SHG response compared with other reported compounds, thereby bolstering its potential as a NLO crystal.
Birefringence characterization
The birefringence of K2 and K3 crystals was evaluated within the visible wavelength range using a polarizing microscope. Fig. 7a–d show the complete extinction of both K2 and K3 crystals under orthogonally polarized light. The measured crystal thicknesses (d) were found to be 29 μm and 16 μm, corresponding to the optical path differences (R) of 841 nm and 896 nm,50 respectively. Consequently, the birefringence values of K2 and K3 crystals were determined to be 0.029 and 0.056, respectively, across the visible wavelength range. The theoretical birefringent values of the two crystals were also calculated, yielding values of 0.028 and 0.051 for K2 and K3 crystals (Fig. 7g and h), respectively, at a wavelength of 1 μm, which aligns with the experimental observations. Notably, the birefringence value of K3 is significantly higher than that of K2, nearly double that of K2. This significant difference primarily stems from the angles between the [BO3] planes in K2 and K3, as discussed in the “Crystal structure” section. Specifically, the spatial structure of K2 consists of two distinct perpendicular [BO3] units oriented at a 90 degree angle to each other, indicating relatively small structural anisotropy. In contrast, K3 displays an arrangement in which the [BO3] units form varying angles, including 63.312, 89.061, and 69.942 degrees, thereby deviating from perpendicularity. This distinct structural feature in K3 results in relatively large structural anisotropy.29
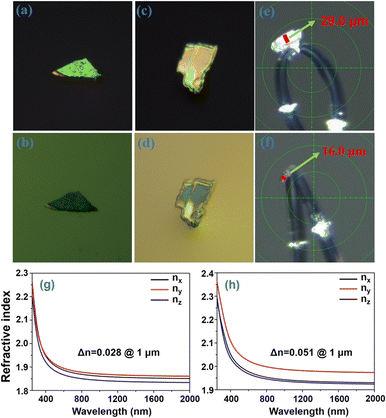 |
| Fig. 7 (a–d) Original interference state and complete extinction of K2 and K3 crystals under cross-polarized light, respectively. (e and f) The thickness of the K2 and K3 crystals, respectively. (g and h) Theoretical dispersion curve of the refractive index for K2 and K3 crystals, respectively. | |
Structure–property correlations
To delve deeper into the electronic structures of K2 and K3 crystals and their inherent relationships with optical properties, this study employed DFT-based computational methods for theoretical research.51 Through first-principles calculations,52 we discovered that both K2 and K3 crystals are direct bandgap compounds, indicating that the lowest point of the conduction band and the highest point of the valence band overlap in momentum space (k-space). The calculated theoretical bandgap values for K2 and K3 crystals are 4.634 eV and 4.001 eV, respectively, as shown in Fig. 8a–c. The orbital contributions of each atom to the energy bands of K2 and K3 can be identified from the corresponding partial densities of states (PDOSs). As shown in Fig. 8b–d, below the Fermi level, the valence band maximum predominantly consists of B 2p and O 2p orbitals, while the conduction band minimum primarily originates from the La 5d orbitals in both K2 and K3 compounds. It is noteworthy that the electron states in K2 predominantly occupied by K 3p orbitals range from −12 to −11 eV, whereas those in K3, governed by K 3p orbitals, span from −13 to −11 eV. Given the close correlation between the optical properties and the optical transitions occurring between electronic states in proximity to the band gap, it can be deduced that the optical activities of the K2 and K3 crystals are primarily influenced by the [BO3] units and [LaO9] polyhedra.
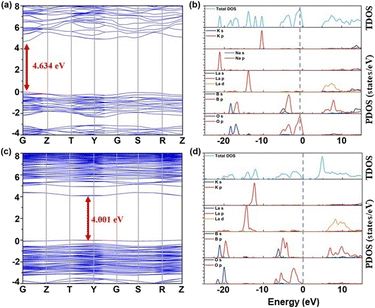 |
| Fig. 8 (a and b) The presentation of the band structure as well as the PDOS and TDOS for K2, respectively. (c and d) The presentation of the band structure as well as the PDOS and TDOS for K3, respectively. | |
Conclusions
In summary, a new family of alkaline-metal rare-earth borates, KxNa3−xLa2B3O9 (x = 2–3), was synthesized through A-site cation control engineering. The investigation of these compounds via DTA-TG analyses and PXRD tests revealed a structural transition from the Pnc2 to the Amm2 space group. Notably, the structural phase of the KxNa3−xLa2B3O9 compounds was found to be modulated by adjusting the A-site cation K+ content. Intriguingly, these materials display large SHG intensities, which are 1.3 to 3.3 times that of KDP with phase-matchable ability, along with short UV absorption edges at approximately 204–208 nm. Moreover, the K2NaLa2B3O9 and K3La2B3O9 crystals selected for analysis possess moderate birefringence values of 0.029 and 0.056, respectively, with the variation primarily attributed to differing orientations of the [BO3] planes. These favorable findings make K2NaLa2B3O9 and K3La2B3O9 good potential candidates for UV NLO applications. The rational regulation of A-site cations underscores a versatile approach for the fabrication of novel NLO crystals with desired performance attributes.
Data availability
The data supporting this article have been included as part of the ESI.† Crystallographic data for 2374500 and 2374501 have been deposited at the CCDC.
Author contributions
Jie Song and Huijian Zhao: experiment, investigation, data curation, writing original draft. Conggang Li: conceptualization, funding acquisition, methodology, project administration, review & editing. Ning Ye, Zhanggui Hu and Yicheng Wu: resources, funding acquisition.
Conflicts of interest
There are no conflicts to declare.
Acknowledgements
This work was supported by the National Key R&D Program of China (2021YFA0717800), National Natural Science Foundation of China (No. 61835014 and 52002273), and State Key Laboratory of Crystal Materials, Shandong University (No. KF2303).
Notes and references
- D. F. Eaton, Nonlinear Optical Materials, Science, 1991, 253, 281–287 CrossRef.
- M. J. Xia, X. X. Jiang, Z. S. Lin and R. K. Lit, “All-Three-in-One”: A New Bismuth-Tellurium-Borate Bi3TeBO9 Exhibiting Strong Second Harmonic Generation Response, J. Am. Chem. Soc., 2016, 138, 14190–14193 CrossRef PubMed.
- X. L. Chen, B. B. Zhang, F. F. Zhang, Y. Wang, M. Zhang, Z. H. Yang, K. R. Poeppelmeier and S. L. Pan, Designing an Excellent Deep-Ultraviolet Birefringent Material for Light Polarization, J. Am. Chem. Soc., 2018, 140, 16311–16319 CrossRef.
- P. S. Halasyamani and J. M. Rondinelli, The must-have and nice-to-have experimental and computational requirements for functional frequency doubling deep-UV crystals, Nat. Commun., 2018, 9, 2972 CrossRef PubMed.
- C. Wu, C. B. Jiang, G. F. Wei, X. X. Jiang, Z. J. Wang, Z. S. Lin, Z. P. Huang, M. G. Humphrey and C. Zhang, Toward Large Second-Harmonic Generation and Deep-UV Transparency in Strongly Electropositive Transition Metal Sulfates, J. Am. Chem. Soc., 2023, 145, 3040–3046 CrossRef PubMed.
- G. H. Zou and K. M. Ok, Novel ultraviolet (UV) nonlinear optical (NLO) materials discovered by chemical substitution-oriented design, Chem. Sci., 2020, 11, 5404–5409 RSC.
- C. Y. Pan, X. R. Yang, L. Xiong, Z. W. Lu, B. Y. Zhen, X. Sui, X. B. Deng, L. Chen and L. M. Wu, Solid-State Nonlinear Optical Switch with the Widest Switching Temperature Range Owing to Its Continuously Tunable Tc, J. Am. Chem. Soc., 2020, 142, 6423–6431 CrossRef.
- N. Zaitseva, L. Carman and I. Smolsky, Habit control during rapid growth of KDP and DKDP crystals, J. Cryst. Growth, 2002, 241, 363–373 CrossRef.
- K. Miyazaki, H. Sakai and T. Sato, Efficient deep-ultraviolet generation by frequency doubling in β-BaB2O4 crystals, Opt. Lett., 1986, 11, 363–373 CrossRef.
- Q. Wang, F. Yang, X. Wang, J. Zhou, J. Ju, L. Huang, D. J. Gao, J. Bi and G. H. Zou, Deep-Ultraviolet Mixed-Alkali-Metal Borates with Induced Enlarged Birefringence Derived from the Structure Rearrangement of the LiB3O5, Inorg. Chem., 2019, 58, 5949–5955 CrossRef.
- B. Wu, D. Tang, N. Ye and C. Chen, Linear and nonlinear optical properties of the KBe2BO3F2 (KBBF) crystal, Opt. Mater., 1996, 5, 105–109 CrossRef.
- Y. Mori, I. Kuroda, S. Nakajima, T. Sasaki and S. Nakai, New nonlinear optical crystal: Cesium lithium borate, Appl. Phys. Lett., 1995, 67, 1818–1820 CrossRef.
- L. Kang and Z. S. Lin, Deep-ultraviolet nonlinear optical crystals: concept development and materials discovery, Light: Sci. Appl., 2022, 11, 201 CrossRef CAS.
- G. A. Valdivia-Berroeta, E. W. Jackson, K. C. Kenney, A. X. Wayment, I. C. Tangen, C. B. Bahr, S. J. Smith, D. J. Michaelis and J. A. Johnson, Designing Non-Centrosymmetric Molecular Crystals: Optimal Packing May Be Just One Carbon Away, Adv. Funct. Mater., 2020, 30, 1904786 CrossRef.
-
(a) M. Mutailipu, K. R. Poeppelmeier and S. L. Pan, Borates: A Rich Source for Optical Materials, Chem. Rev., 2021, 121, 1130–1202 CrossRef PubMed;
(b) M. Mutailipu, M. Zhang, H. P. Wu, Z. H. Yang, Y. H. Shen, J. L. Sun and S. L. Pan, Ba3Mg3 (BO3)3F3 polymorphs with reversible phase transition and high performances as ultraviolet nonlinear optical materials, Nat. Commun., 2018, 9, 3809 CrossRef;
(c) X. Long, R. An, Y. Lv, X. Wu and M. Mutailipu, Tunable Optical Anisotropy in Rare-Earth Borates with Flexible [BO3] Clusters, Chem.–Eur. J., 2024, 30, e202401488 CrossRef PubMed.
- J. L. Song, C. L. Hu, X. Xu, F. Kong and J. G. Mao, A Facile Synthetic Route to a New SHG Material with Two Types of Parallel π-Conjugated Planar Triangular Units, Angew. Chem., Int. Ed., 2015, 54, 3679–3682 CrossRef PubMed.
- H. Huppertz and B. von der Eltz, Multianvil high-pressure synthesis of Dy4B6O15: the first oxoborate with edge-sharing BO4 tetrahedra, J. Am. Chem. Soc., 2002, 124, 9376–9377 CrossRef PubMed.
- G. Sohr, N. Ciaghi, M. Schauperl, K. Wurst, K. R. Liedl and H. Huppertz, High-Pressure Synthesis of Cd(NH3)2[B3O5(NH3)]2: Pioneering the Way to the Substance Class of Ammine Borates, Angew. Chem., Int. Ed., 2015, 54, 6360–6363 CrossRef PubMed.
- F. Kong, S. P. Huang, Z. M. Sun, J. G. Mao and W. D. Cheng, Se2(B2O7): a new type of second-order NLO material, J. Am. Chem. Soc., 2006, 128, 7750–7751 CrossRef PubMed.
-
(a) W. Q. Lu, Z. L. Gao, X. T. Liu, X. X. Tian, Q. Wu, C. G. Li, Y. X. Sun, Y. Liu and X. T. Tao, Rational Design of a LiNbO3-like Nonlinear Optical Crystal, Li2ZrTeO6, with High Laser-Damage Threshold and Wide Mid-IR Transparency Window, J. Am. Chem. Soc., 2018, 140, 13089–13096 CrossRef PubMed;
(b) C. F. Sun, C. L. Hu, X. Xu, J. B. Ling, T. Hu, F. Kong, X. F. Long and J. G. Mao, BaNbO(IO3)5: A New Polar Material with a Very Large SHG Response, J. Am. Chem. Soc., 2009, 131, 9486 CrossRef PubMed.
-
(a) H. Chen, W. B. Wei, H. Lin and X. T. Wu, Transition-metal-based chalcogenides: a rich source of infrared nonlinear optical materials, Coord. Chem. Rev., 2021, 448, 214154 CrossRef;
(b) C. G. Li, Z. L. Gao, P. Zhao, X. X. Tian, H. Y. Wang, Q. Wu, W. Q. Lu, Y. X. Sun, D. L. Cui and X. T. Tao, Crystallographic Investigations into the Polar Polymorphism of BaTeW2O9: Phase Transformation, Controlled Crystallization, and Linear and Nonlinear Optical Properties, Cryst. Growth Des., 2019, 19, 1767–1777 CrossRef CAS.
- Y. N. Chen, M. Zhang, M. Mutailipu, K. R. Poeppelmeier and S. L. Pan, Research and Development of Zincoborates: Crystal Growth, Structural Chemistry and Physicochemical Properties, Mol, 2019, 24, 2763 CrossRef.
-
(a) D. Schultze, K.-T. Wilke and C. Waligora, Zur Chemie in Schmelzlösungen. IV. Darstellung von kristallinen Boraten M2+M4+(BO3)2, Z. Anorg. Allg. Chem., 1971, 380, 37–40 CrossRef CAS;
(b) V. Hornebecq, P. Gravereau, J. P. Chaminade and E. Lebraud, BaZr(BO3)2: a non-centrosymmetric dolomite-type superstructure, Mater. Res. Bull., 2002, 37, 2165–2178 CrossRef CAS.
- A. Akella and D. A. Keszler, Crystal Chemistry of Noncentrosymmetric Alkali-Metal Nb and Ta Oxide Pyroborates, J. Solid State Chem., 1995, 120, 74–79 CrossRef.
- J. Choisnet, D. Groult, B. Raveau and M. Gasperin, Nouvelles structures à tunnels de section pentagonale K3Nb3B2O12 et K3Ta3B2O12, Acta Crystallogr., 1977, 33, 1841–1845 CrossRef.
- K. Zhang, X. Chen and X. Wang, Review of Study on Bismuth Triborate (BiB3O6) Crystal, J. Synth. Cryst., 2005, 34, 438 Search PubMed.
- J. Barbier and L. M. D. Cranswick, The non-centrosymmetric borate oxides, MBi2B2O7 (M = Ca, Sr), J. Solid State Chem., 2006, 179, 3958–3964 CrossRef.
- W. L. Zhang, W. D. Cheng, H. Zhang, L. Geng, C. S. Lin and Z. Z. He, A Strong Second-Harmonic Generation Material Cd4BiO(BO3)3 Originating from 3-Chromophore Asymmetric Structures, J. Am. Chem. Soc., 2010, 132, 1508 CrossRef PubMed.
- M. Mutailipu, F. M. Li, C. C. Jin, Z. H. Yang, K. R. Poeppelmeier and S. L. Pan, Strong Nonlinearity Induced by Coaxial Alignment of Polar Chain and Dense BO3 Units in CaZn2(BO3)2, Angew. Chem., Int. Ed., 2022, 61, e202202096 CrossRef CAS PubMed.
- Y. Q. Chen, J. K. Liang, Y. X. Gu, J. Luo, J. B. Li and G. H. Rao, Synthesis and crystal structure of a novel hexaborate, Na2ZnB6O11, Powder Diffr., 2010, 25, 9–14 CrossRef CAS.
- H. W. Yu, H. P. Wu, S. L. Pan, Z. H. Yang, X. L. Hou, X. Su, Q. Jing, K. R. Poeppelmeier and J. M. Rondinelli, Cs3Zn6B9O21: A Chemically Benign Member of the KBBF Family Exhibiting the Largest Second Harmonic Generation Response, J. Am. Chem. Soc., 2014, 136, 1264–1267 CrossRef CAS PubMed.
- X. H. Meng, X. Y. Zhang, Q. X. Liu, Z. Y. Zhou, X. X. Jiang, Y. G. Wang, Z. S. Lin and M. J. Xia, Perfectly Encoding π-Conjugated Anions in the RE5(C3N3O3)(OH)12 (RE = Y, Yb, Lu) Family with Strong Second Harmonic Generation Response and Balanced Birefringence, Angew. Chem., Int. Ed., 2023, 62, e202214848 CrossRef CAS PubMed.
- Z. H. Yang, A. Tudi, B. H. Lei and S. L. Pan, Enhanced nonlinear optical functionality in birefringence and refractive index dispersion of the deep-ultraviolet fluorooxoborates, Sci. China Mater., 2020, 63, 1480–1488 CrossRef CAS.
- Y. Wang, B. B. Zhang, Z. H. Yang and S. L. Pan, Cation-Tuned Synthesis of Fluorooxoborates: Towards Optimal Deep-Ultraviolet Nonlinear Optical Materials, Angew. Chem., Int. Ed., 2018, 57, 2150–2154 CrossRef CAS.
- M. Mutailipu, Z. Q. Xie, X. Su, M. Zhang, Y. Wang, Z. H. Yang, M. Janjua and S. L. Pan, Chemical Cosubstitution-Oriented Design of Rare-Earth Borates as Potential Ultraviolet Nonlinear Optical Materials, J. Am. Chem. Soc., 2017, 139, 18397–18405 CrossRef PubMed.
- Y. F. Li, F. Liang, H. M. Song, W. Liu, Z. S. Lin, G. C. Zhang and Y. C. Wu, Rb7SrY2(B5O10)3: A Rare-Earth Pentaborate with Moderate Second-Harmonic Response and Interesting Phase-Matching Behavior, Inorg. Chem., 2019, 58, 8943–8947 CrossRef.
- G. C. Zhang, Y. C. Wu, P. Z. Fu, G. F. Wang, S. L. Pan and C. T. Chen, A new nonlinear optical berate crystal Na3La2(BO3)3, Chem. Lett., 2001, 30, 456–457 CrossRef.
- J. Song, C. G. Li, J. M. Jiao, Y. H. She, W. L. Zhao, F. Liang, N. Ye, Z. G. Hu and Y. C. Wu, KNa2La2(BO3)3: a shortite-type lanthanide borate exhibiting strong nonlinear optical activity induced by isolated BO3 triangles and distorted LaO9 polyhedra, Inorg. Chem. Front., 2023, 10, 5488–5495 RSC.
- H. N. Liu, H. P. Wu, Z. G. Hu, J. Y. Wang, Y. C. Wu, P. S. Halasyamani and H. W. Yu, Rb3B11P2O23: Materials Design of a New Chemically Benign Deep-Ultraviolet Nonlinear Optical Material, ACS Mater. Lett., 2023, 5, 155–161 CrossRef.
- H. A. Liu, H. P. Wu, Z. G. Hu, J. Y. Wang, Y. C. Wu and H. W. Yu, Cs3(BOP)2(B3O7)3: A Deep-Ultraviolet Nonlinear Optical Crystal Designed by Optimizing Matching of Cation and Anion Groups, J. Am. Chem. Soc., 2023, 145, 12691–12700 CrossRef PubMed.
- X. M. He, L. Qi, W. Y. Zhang, R. X. Zhang, X. Y. Dong, J. H. Ma, M. Abudoureheman, Q. Jing and Z. H. Chen, Controlling the Nonlinear Optical Behavior and Structural Transformation with A-Site Cation in α-AZnPO4 (A = Li, K), Small, 2023, 19, 2206991 CrossRef PubMed.
- S. J. Han, Y. Wang, S. L. Pan, X. Y. Dong, H. P. Wu, J. Han, Y. Yang, H. W. Yu and C. Y. Bai, Noncentrosymmetric versus Centrosymmetric: Influence of the Na+ Substitution on Structural Transition and Second-Harmonic Generation Property, Cryst. Growth Des., 2014, 14, 1794–1801 CrossRef.
-
(a) Y. C. Wu, J. G. Liu, P. Z. Fu, J. X. Wang, H. Y. Zhou, G. F. Wang and C. T. Chen, A new lanthanum and calcium borate La2CaB10O19, Chem. Mater., 2001, 13, 753–755 CrossRef;
(b) R. Q. Liu, H. P. Wu, H. W. Yu, Z. G. Hu, J. Y. Wang and Y. C. Wu, K5Mg2La3(BO3)6: An Efficient, Deep-Ultraviolet Nonlinear Optical Material, Chem. Mater., 2021, 33, 4240–4246 CrossRef;
(c) Z. Q. Xie, M. Mutailipu, G. J. He, G. P. Han, Y. Wang, Z. H. Yang, M. Zhang and S. L. Pan, A Series of Rare-Earth Borates K7MRE2B15O30 (M = Zn, Cd, Pb; RE = Sc, Y, Gd, Lu) with Large Second Harmonic Generation Responses, Chem. Mater., 2018, 30, 2414–2423 CrossRef.
- L. L. H awes, The determination of lattice constants using low angle diffraction lines, Acta Crystallogr., 1959, 12, 443–445 CrossRef.
- P. Kubelka and F. Munk, An Article on Optics of Paint Layers, Zeitschrift für Technische Physik, 1931, 12, 593–601 Search PubMed.
- F. He, J. H. Zhuang, B. Lu, X. L. Liu, J. L. Zhang, F. N. Gu, M. H. Zhu, J. Xu, Z. Y. Zhong, G. W. Xu and F. B. Su, Ni-based catalysts derived from Ni-Zr-Al ternary hydrotalcites show outstanding catalytic properties for low-temperature CO2 methanation, Appl. Catal., B, 2021, 293, 120218 CrossRef.
- S. K. Kurtz and T. T. Perry, A Powder Technique for the Evaluation of Nonlinear Optical Materials, J. Appl. Phys., 1968, 39, 3798–3813 CrossRef.
- X. Y. Li, Q. Wei, C. L. Hu, J. Pan, B. X. Li, Z. Z. Xue, X. Y. Li, J. H. Li, J. G. Mao and G. M. Wang, Achieving Large Second Harmonic Generation Effects via Optimal Planar Alignment of Triangular Units, Adv. Funct. Mater., 2023, 33, 2210718 CrossRef.
-
(a) G. C. Zhang, Y. C. Wu, Y. G. Li, F. Chang, S. L. Pan, P. Z. Fu and C. T. Chen, Flux growth and characterization of a new oxyborate crystal Na3La9O3(BO3)8, J. Cryst. Growth, 2005, 275, E1997–E2001 CrossRef CAS;
(b) M. E. Gao, H. P. Wu, H. W. Yu, Z. G. Hu, J. Y. Wang and Y. C. Wu, BaYOBO3: a deep-ultraviolet rare-earth oxy-borate with a large second harmonic generation response, Sci. China: Chem., 2021, 64, 1184–1191 CrossRef CAS;
(c) F. X. Shan, L. Kang, G. C. Zhang, J. Y. Yao, Z. S. Lin, M. J. Xia, X. Y. Zhang, Y. Fu and Y. C. Wu, Na3Y3(BO3)4: a new noncentrosymmetric borate with an open-framework structure, Dalton Trans., 2016, 45, 7205–7208 RSC;
(d) F. X. Shan, M. J. Xia, G. C. Zhang, J. Y. Yao, X. Y. Zhang, T. X. Xu and Y. C. Wu, Growth, structure, and optical properties of a self-activated crystal: Na3Nd9O3(BO3)8, Solid State Sci., 2015, 41, 31–35 CrossRef;
(e) R. A. Kumar, M. Arivanandhan and Y. Hayakawa, Recent advances in rare earth-based borate single crystals: potential materials for nonlinear optical and laser applications, Prog. Cryst. Growth Charact. Mater., 2013, 59, 113–132 CrossRef;
(f) M. Iwai, T. Kobayashi, H. Furuya, Y. Mori and T. Sasaki, Crystal Growth and Optical Characterization of Rare-Earth (Re) Calcium Oxyborate ReCaO(BO)(Re = Y or Gd) as New Nonlinear Optical Material, Jpn. J. Appl. Phys., 1997, 36, L276–L279 CrossRef;
(g) Y. Q. Liu, F. P. Yu, Z. P. Wang, S. Hou, L. Yang, X. G. Xu and X. Zhao, Bulk growth and nonlinear optical properties of thulium calcium oxyborate single crystals, Crystengcomm, 2014, 16, 7141–7148 RSC.
- W. Q. Huang, X. Zhang, Y. Q. Li, Y. Zhou, X. Chen, X. Q. Li, F. F. Wu, M. C. Hong, J. H. Luo and S. G. Zhao, A Hybrid Halide Perovskite Birefringent Crystal, Angew. Chem., Int. Ed., 2022, 61, e202202746 CrossRef PubMed.
- R. W. Godby, M. Schlüter and L. J. Sham, Self-energy operators and exchange-correlation potentials in semiconductors, Phys. Rev. B: Condens. Matter Mater. Phys., 1988, 37, 10159–10175 CrossRef.
- S. J. Clark, M. D. Segall, C. J. Pickard, P. J. Hasnip, M. J. Probert, K. Refson and M. C. Payne, First principles methods using CASTEP, Z. Kristallogr., 2005, 220, 567–570 Search PubMed.
Footnotes |
† Electronic supplementary information (ESI) available: Experimental section, CIF file, crystallographic data, IR spectra, and SHG comparison for K2 and K3. CCDC 2374500 and 2374501 for K2 and K3. For ESI and crystallographic data in CIF or other electronic format see DOI: https://doi.org/10.1039/d4sc05081a |
‡ Song J. and Zhao H. contributed equally to this work. |
|
This journal is © The Royal Society of Chemistry 2024 |
Click here to see how this site uses Cookies. View our privacy policy here.